All published articles of this journal are available on ScienceDirect.
Hydrogen Oxidizing Bacteria as Novel Protein Source for Human Consumption: An Overview
Abstract
The increasing threat of climate change combined with the prospected growth in the world population puts an enormous pressure on the future demand for sustainable protein sources for human consumption. In this review, hydrogen oxidizing bacteria (HOB) are presented as a novel protein source that could play a role in fulfilling this future demand. HOB are species of bacteria that merely require an inflow of the gasses hydrogen, oxygen, carbon dioxide, and a nitrogen source to grow in a conventional bioreactor. Cupriavidus necator is proposed as HOB for industrial cultivation due to its remarkably high protein content (up to 70% of mass), suitability for cultivation in a bioreactor, and the vast amount of available background information. A broad overview of the unique aspects of the bacteria will be provided, from the production process, amino acid composition, and source of the required gasses to the future acceptance of HOB into the market.
1. INTRODUCTION
With a rapidly growing world population and the increasing severity of the effects of climate change, the need for (novel) protein sources will become ever imminent. As a result, alternative protein sources, such as algal or bacterial proteins, have been gaining popularity quickly. HOBs show great potential as a sustainable protein source produced from hydrogen gas obtained from the electrolysis of water. This review displays the possibilities and challenges the use of HOB as a human protein source.
The world faces three main problems regarding the future protein production: Climate change, the preservation of our planet, and population growth [1]. This growth, combined with rising income levels, will increase the demand for animal protein [2, 3]. To be able to ensure food and feed security, crop production must be expanded significantly. However, climate change makes this expansion more difficult due to, for example, rising temperatures, extreme weather conditions and increased droughts [2]. Both yield and nutritional value of crops will likely be affected [4].
In 2017, the contribution of agriculture to all greenhouse gas emissions was 20% [5]. Meat is especially large contributor: of all proteins produced from meat, only 10% ends up in the final product due to leaky supply chains or consumer preferences [6, 7]. An increased use of plant protein sources could reduce the total greenhouse gas emissions significantly while increasing the protein yield. However, agriculture has been proven to leak 50-70% of its nitrogen to the surrounding environment, which suggests that agricultural protein has a relatively large footprint as well [8].
The EAT Lancet report of 2019 takes the earth’s boundaries and population growth into consideration and has developed guidelines to provide a healthy and sustainable diet for a world population of up to 10 billion people by 2050, which is compatible with the UN population projection [9]. In this report, it is said that to be able to achieve this goal, the food production and consumption system needs to be radically changed. Regarding the sustainability of food production, the report states: “sustainable food production for about 10 billion, people should use no additional land, safeguard existing biodiversity, reduce consumptive water use and manage water responsibly, substantially reduce nitrogen and phosphorus pollution, produce zero carbon dioxide emissions, and cause no further increase in methane and nitrous oxide emissions” [9].
According to UN population projections, the world population will be around 9.7 billion in 2050 [10]. To be able to provide a fast-growing population with a healthy protein-rich diet, the production of protein will need to be increased by 100-110% in 2050 in comparison to 2005 [11]. This burden cannot fall solely on meat or agricultural protein due to both limitations in sustainability and the availability of fertile land.
Hence, the world calls for a more sustainable way of protein production for human consumption. Single cell protein (SCP) like algae, fungi and bacteria have potential to fulfil this role [12]. Even though SCPs, including HOB, have been studied for years, the available SCP-protein products for human consumption are scarce [12-26]. The study of Anupama [12] reviews the limitations of algae, fungi and bacteria as protein sources for human consumption. It concludes that each source has their own limitations concerning digestibility, health issues and production costs. These factors explain the current scarcity of SCP-protein for human consumption.
HOB are a polyphyletic group of bacteria that use hydrogen gas as an energy source and electron donor, oxygen as an electron acceptor, ammonia as a nitrogen source, and carbon dioxide as a carbon source. This means these bacteria can be grown in a stirred reactor on the basis of the supply of H2, O2, CO2 and NH3. The hydrogen gas will be acquired by electrolysis, powered by solar or wind energy. The aim would be to render this process fully neutral in climate impact. The production process of HOB is schematically displayed in Fig. (1).
Therefore, HOB could play a role in eradicating hunger worldwide by removing the pressure on agricultural lands, offering consistent “harvests” and having little impact on climate change. It is already used as an ingredient in feed [25]. Additionally, HOB-protein product is in development to be used in food products as an ingredient, currently being done with whey and soy protein. The preference lies with human nutrition since feeding livestock with high quality protein is a waste of resources. This is confirmed by the study of Pikaar et al. [27], who stated that the nitrogen consumed from plant-based products was 14%, animal products 4%, animal products with SCP 10% and SCP food 43%.
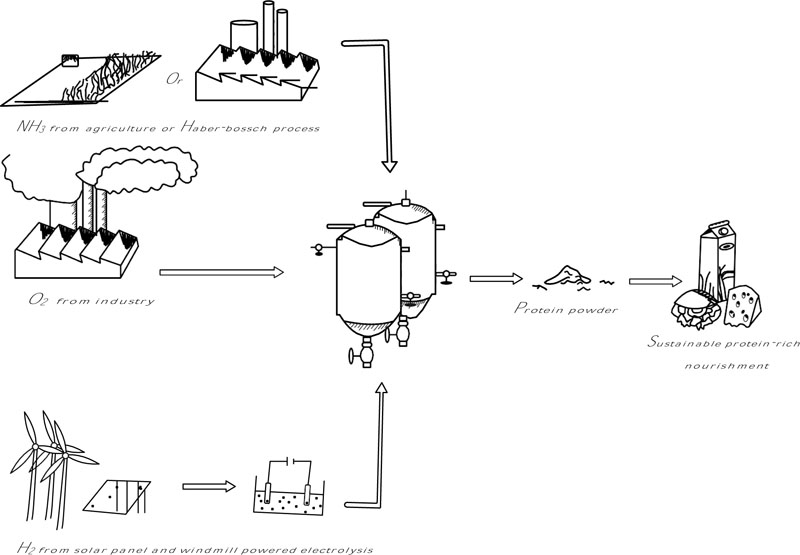
This review displays an extensive overview of HOB as an alternative protein source regarding the different HOB species, the chemical mechanisms that occur in these bacteria, the process of technological production, sourcing, the protein composition and possible future applications. In addition, an outline will be given on the public view towards bacteria for food consumption and a marketing approach to increase public acceptance.
2. AEROBIC HYDROGEN OXIDIZING BACTERIA AS SINGLE CELL PROTEIN SOURCE
The concept of using microorganisms as a source of food and feed is at least several centuries old. Examples are dating to the 16th century in Mexico, S. maxima was harvested from Lake Texcoco, dried, and sold for human consumption [28]. The use of hydrogen-oxidizing chemoautotrophic bacteria for the production of edible microbial biomass from CO2 was already proposed several decades ago as a circular food production system for space travel [29].
Hydrogen oxidizing bacteria, also called “Knallgas” bacteria, named after the gaseous mixture of oxygen and hydrogen, are a polyphyletic group of facultative chemoautotrophic bacteria [20, 30-32]. In the study of Schink and Schlegel [33], heterogenous groups of taxa were identified, such as Alcaligenes, Pseudomonas, Paracoccus, Aquaspirilium, Flavobacterium, Corynebacterium, Nocardia, Mycobacterium, and Bacillus. These bacteria are able to fix carbon dioxide into new cellular material via the ribulose biphosphate or reverse tricarboxylic cycle. HOB can contain high amounts of protein, up to 70% of the dry weight [20]. This protein is characterised by an amino acid profile more similar to high-quality animal protein than vegetable proteins, which is further discussed in the 'qualitative protein analysis' [34].
The majority of HOB is not able to fixate the molecular nitrogen present in air, but needs a fixed form of nitrogen. However, there is a small group of HOB which is also able to fix molecular nitrogen and therefore does not need a form of fixed nitrogen. Xanthobacter autotrophicus is the best-known species with this ability and has also been tested as a bio-fertilizer [35, 36]. For the use of nitrogen fixing HOB as a single cell protein (SCP) [24], has grown several species. These species could grow without ammonium in the medium and yielded 50-80% of the biomass from cultures with ammonium in the medium. The protein content of the bacteria without reactive mineral nitrogen was about 10% lower than that of bacteria grown with it. However, the essential amino acid profile is similar to bacteria grown on reactive mineral nitrogen [13]. Although the lower yield makes it less attractive, it could, in some cases, be attractive because of the environmental impact of nitrogen fixation.
2.1. Cupriavidus Necator
Although HOBS are omnipresent, they have received relatively little attention, especially as a protein source for human consumption. To be sold for human consumption within the European Union, the bacterial protein has to be accepted by the European Food Safety Authority. One of the rules is that the bacterium has to grow in pure culture. Because of this, we review a single species rather than mixotrophic cultures. A HOB with a high potential for this production process is Cupriavidus necator. It has a protein content of up to 70% of the dry weight and a high-quality amino acid profile [14, 20]. This is also the best-described species of HOB in literature, taking into account the different names the species has had (Alcaligenes eutropha, Hydrogenomonas eutrophus, and Wautersia eutropha). The full genomes have been sequenced for the H16 strain [37, 38] and for the JMP134 strain [39]. All together, this seems to be the most suited strain for the near future.
C. necator is mainly known for its ability to produce polyhydroxyalkanoates, and it is the model organism for this type of metabolism [40]. Polyhydroxybutyric acid is formed under autotrophic growth and nutrient limiting conditions [41].
2.2. Optimal Growth Condition of C. necator for High Protein Yield
The ratio of the gas mixture greatly influences the growth rate of the bacteria. Since a mixture of hydrogen and oxygen gas can easily explode, the preferences of the bacteria and safety must be taken into account. For oxygen, the lower explosion limit is 6 vol% in excess of hydrogen gas [42]. Fortunately, 4 vol% O2 in the gas phase was found to be optimal for the growth of C. necator [43].
For the growth rate, a maximal growth rate of 0.12 h.-1 was found by Yu and Lu [44], in an experimental study. This was at 30°C and with H2:O2:CO2 equaling 7:2:1 in the gas phase. A reaction heat of 1764.9 kJ C.mol−1 was observed until a density of 7.7g/L was reached for the dry weight. In the exponential phase, the following equation is applied for the growth of the bacteria:
CO2 + 7.77H2 + 2.87O2 + 0.24NH3 → CH1.68O0.46N0.24 + 7.28H2O
The energy efficiency of electrical energy from biomass was found to be 6,4% under autotrophic conditions [45]. In comparison, for microalgae, maximal energy efficiency of 4-6% was found to go from electrical energy to biomass [46].
As shown above, the maximum growth rate of 0.12 h.-1 can be obtained under ideal conditions. However, if nutrients are not supplied in the right ratio, the microbe will not develop protein or will grow slower. Carbon dioxide concentration is an important factor in the growth rate of bacteria [47]. If CO2 limiting conditions occur, the hydrogen efficiency will decrease drastically because the bacteria will not have enough carbon available to convert to cellular material [45]. Another factor for growth is nitrogen concentration, which has a big impact on the specific growth rate. For the bacterium C. eutrophus, a species related to C. necator, the specific growth rate drops more than 30% when 50% of the necessary nitrogen is available [48]. If nitrogen-limiting conditions occur, the carbon will be used for the formation of PHB, which is not a protein [47].
3. OXYGEN RESISTANCE MECHANISM AND ITS EFFECTS
There are aerobic and anaerobic hydrogen oxidizing bacteria. Both aerobic and anaerobic HOBS have hydrogenase enzymes to process H2 gas for growth. Hydrogenases of the anaerobic HOB species are irreversibly inhibited under even trace amounts of molecular oxygen (O2), which prevents bacterial growth in an oxygenated environment. In contrast, C. necator, which belongs to the aerobic HOB, can grow under dissolved oxygen concentrations up to 40% [49]. The optimal growth condition of this bacterium is at 4.8 vol% dissolved oxygen [43]. Growth under these conditions is possible because C. necator has unique hydrogenase enzymes that are resistant to inhibition by oxygen. Because of the oxygen-resistant hydrogenases, C. necator can use aerobic respiration, which is more efficient than anaerobic respiration. As a result, more energy for growth can be obtained from one H2 molecule. The combination of H2 dependent growth with aerobic respiration is rare and is made possible by adjustments in the hydrogenase of C. necator. By studying the hydrogenases, the origin of the oxygen resistance can be determined.
3.1. The Hydrogenases of C. necator
Hydrogenases are enzymes which catalyse the cleavage of H2 into hydrogen ions (H+) and electrons. The electrons from H2 oxidation are used to generate ATP or produce reducing factors like NADH in C. necator [50-52]. C. necator carries four types of hydrogenases, but one of the hydrogenases is of no interest to this chapter due to its inactivity in the lithoautotrophic pathway [53]. The three that are relevant are regulatory hydrogenase (RH), membrane-bound hydrogenase (MBH), and soluble hydrogenase (SH) [52, 54, 55]. The active site of all three enzymes contains a nickel-iron ([Ni-Fe]) metal complex that acts as the catalyst. Via redox reactions, this metal group can convert H2 to separate hydrogen ions and electrons [56]. Oxygen can also bind to the [Ni-Fe] catalyst, which deactivates the enzyme. To survive in an oxygen-rich environment, all these three hydrogenases need to be modified to withstand oxidation by O2. This is done in a different way for each hydrogenase, which will be elaborated on accordingly.
3.2. Oxygen Resistance of Hydrogenases
The RH is located in the cytoplasm of C. necator and functions as a hydrogen sensor [54, 57]. When there is H2 present, the RH activates regulators, which promote gene transcription of the MBH and SH operons [54]. The oxygen resistance of the RH is based on the access of O2 to the active site. Special gas channels in the amino acid sequence prevent oxygen from reaching the active site by using bulky amino acid residues that make the channels too narrow for oxygen. The smaller H2 molecule is guided through these channels to the active site [58].
The MBH is located at the outside of the cell membrane. It contains a cytochrome b subunit, which transfers electrons from the hydrogenase to the ubiquinone pool of C. necator [50]. The oxygen resistance of MBH can be explained by the presence of a [4Fe-3S] cluster next to the [Ni-Fe] site [59]. This [4Fe-3S] structure is responsible for the reduction of oxygen to water with hydrogen and electrons, as presented in reaction 2. First, four electrons from C. necator are transported to the catalytic centre via a reverse electron flow, where they reduce the bound O2. Subsequent reaction with four protons (H+) forms two H2O molecules that can leave the catalytic centre via special water channels in the enzyme. By reducing the bound oxygen to water at the active site, the enzyme is free again to undergo any subsequent hydrogen cleavage reactions [60]. Binding of oxygen to the metal catalyst temporarily inhibits the activity of the enzyme. Compared to anaerobic conditions, the activity of the isolated MBH hydrogenase is decreased by approximately 40% at an oxygen concentration of 5 vol%. The activity is retained for 40% under a dissolved oxygen concentration of 20 vol% [61].
4H+ + 4e- + O2 ↔ 2 H2O (reaction 2)
In the cytoplasm of C. necator, the SH is located [62]. The catalytic [Ni-Fe] site is connected to a NAD+ reductase subunit with a relay of [4Fe-4S] and [2Fe-S2] complexes, which facilitate electron transport between the two sites [63]. The electrons produced by the hydrogenase are used by the reductase to reduce NAD+ to NADH, which is an important cofactor in the metabolism of living organisms [52]. The oxygen resistance of SH is regulated by multiple different dynamics. The first is the inhibition of O2 diffusion through gas channels by specific amino acid residues, decreasing the amount of oxygen that reaches the active site of the enzyme [64]. Secondly, by reduction of O2 bound to the active site, the SH can free itself, similar to the oxygen-resistant mechanism of MBH. The key difference between them is that SH has two binding sites for oxygen instead of one, the catalytic [Ni-Fe] site and the NAD+ binding site. Additionally, during the reduction of oxygen in the SH, there is not only the production of water but also hydrogen peroxide (H2O2) and low amounts of superoxide (O2-) [65, 66]. These pathways thus ensure the reduction of oxygen bound to the catalytic SH site of C. necator, which allows growth in oxygen-containing environments. In the SH, oxygen present in the environment has only a small effect on enzyme activity. Compared to anaerobic conditions, no significant decrease in activity is visible at an oxygen concentration of 20%. Additionally, the activity of the isolated SH hydrogenase decreases with 20% when 80 vol% oxygen is present. At 40% oxygen, the maximal oxygen concentration for the growth of C. necator, 3% of the electrons produced by H2 oxidation are reversibly used to reduce the oxygen bound to the active site [66].
3.3. Carbon Dioxide Fixation
C. necator has the ability to fixate CO2 from its environment. The Calvin-Benson-Bassham (CBB) cycle is utilized for the fixation of CO2 [16]. C. necator’s H16 strain uses, amongst others, intermediates of the reverse tricarboxylic cycle (rTCA) to support heterotrophic growth [67]. Under autotrophic conditions, C. necator fixes carbon dioxide via the Calvin-Benson-Bassham (CBB) cycle, which is more similar to plant protein, which is derived from plants using the CBB cycle with rubisco as the protein [16]. The bacterium further also has an oxidative-TCA cycle which is more active under mixotrophic conditions, such as when oxidizing hydrogen, than under heterotrophic conditions [68]. In the reductive tricarboxylic acid (rTCA) cycle two molecules of CO2 are used for the biosynthesis of acetyl-CoA. This makes rTCA a reverse process of the oxidative TCA cycle, also known as Krebs cycle. C. necator makes use of the (r)TCA cycle during cultivation in bioreactors [68-71].
4. BIOREACTOR
Hydrogen-oxidizing bacteria (e.g. C. necator) can be grown on recovered carbon dioxide, nitrogen, and electrolytically produced oxygen and hydrogen gas [72]. The conventional method for growing these bacteria is by leading gas mixtures of CO2, H2, and O2 through a single sparger into the bioreactor containing the bacteria [73]. The easiest method for the production of hydrogen gas is water electrolysis, which has an efficiency of 90% [74].
Once all the necessary components have been acquired, the design of the reactor should be considered. The gas transfer towards the liquid phase weighs heavily in this decision, as the solubility of oxygen and hydrogen gas is relatively low compared to the need of the HOB cells [75]. In addition, the total gas composition should stay within the explosion limit. There is an increased explosion risk at oxygen concentrations larger than 4 vol%, whereas the bacteria grow optimally within the explosion range at 6 vol% [42]. However, there are also studies reporting optimal growth conditions at an oxygen concentration of 4 vol%, which is within the explosion limit [43]. The gas distribution and composition are substantial hurdles to overcome for a high biomass yield [47].
There are two ways one could increase the gas transfer towards the liquid phase: Increase the contact area of the gas (thus increasing the kLa) or the concentration of the gas in the liquid phase [42]. The contact area can be increased by the use of specialized impellers [45] or nozzles that increase the liquid circulation [45]. Using a gas vector, gas that can help transport another gas, is also an option for increasing the contact area of the gas. Nevertheless, in a study performed by Yu and Munasinghe [47], this was found to have a little positive effect on the kLa and might damage cell membranes of the HOBS, thereby reducing the growth rate.
An increase in the oxygen concentration in the liquid could be reached by culturing at higher pressures while keeping the gas ratio the same. Then, no changes in sparging or stirring are required. This technique for growing C. necator was successfully proved by Yu and Munasinghe [47]. According to Henry’s law, when the pressure rises, the concentration in the liquid state rises as well and thus increasing the gas flux. When the pressure is raised from 1 atm to 4 atm, 40% more cell mass is formed. Still, the impact of this method on cell viability and productivity during long fermentation runs is unclear.
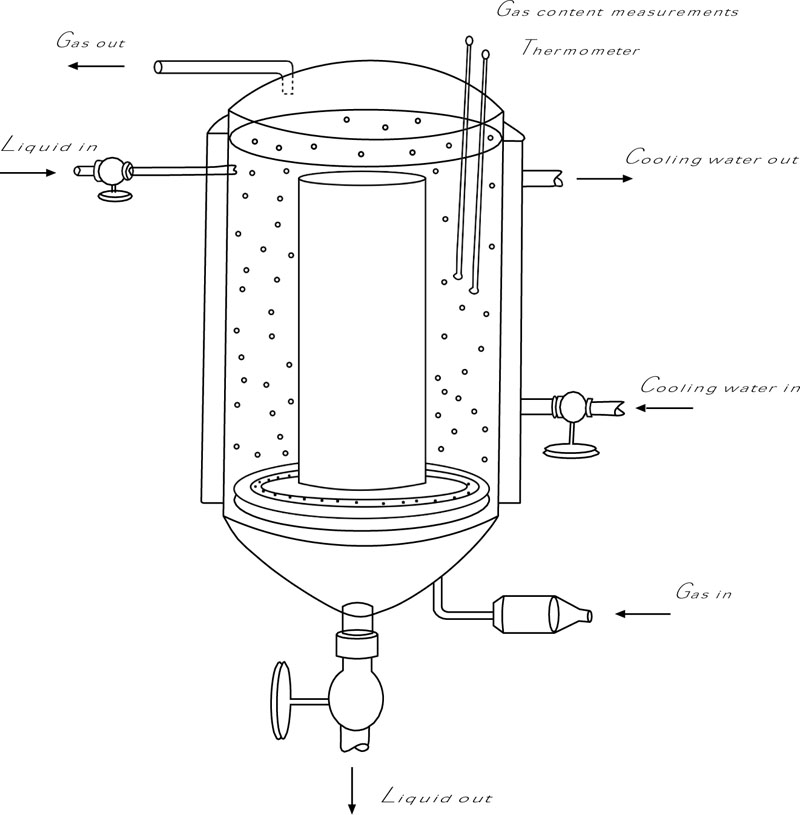
4.1. Bioreactor Design
An airlift reactor has been proposed for the growth of HOBS, taking the latter limitations into consideration. Airlift reactors consist of an aerated riser and a downcomer, indicated in Fig. (2), which induce hydrostatic pressure differences over the tank [76]. A liquid flow over the longitudinal direction of the tank is induced, leading to a decrease in gas hold-ups and improvement in oxygen transfer. A small headspace would be advisable in this case, which would keep the hydrogen and oxygen concentration within the explosion limits (hydrogen: 5-75%) [77].
A good alternative would be a packed bed bioreactor, which consists of a column stacked with solid materials. The liquid phase spreads as a layer over the column, whereas the gas stream moves in the counter direction [78]. Hereby, a larger specific surface is created, allowing for high kOLa values at low gassing rates (< 0.02 vvm) and ideal stream velocities [45]. In addition, the pressure build-up will be considerably lower, meaning less electrical power will be needed to acquire a certain gas velocity.
4.2. Optimization of Electrolysis with Emerging Bioreactors
Most research has been done so far with HOBS in conventional airlift bioreactors and several ways HOBS growth can be optimized have already been discussed. Other, more rigorous, ways of improving mass transfer area, however available, such as the use of Microbial fuel cells (MFCs) and Microbial Electrolysis cells (MECs) [79, 80]. In these cases, hydrogen is produced by microorganisms breaking down e.g., organic matter, resulting in hydrogen yields up to 100% that are directly introduced into the liquid [81]. However, these new methods need organic sources, whereas the principal advantage of hydrogen-oxidizing bacteria is based on their growth in renewable sources and waste streams.
On the other hand, there are emerging technologies researching the use of combined reactors for HOB growth and electrolysis, without the use of extra micro-organisms. These reactors aim to optimize the hydrogen gas production and delivery into the liquid stream and show much potential for the growth of HOBS in waste streams. In this case, HOBS would be grown in a two-chamber cell, which is available in various shapes and sizes. As these techniques are incredibly novel and still under research as well, HOB growth in them is not realistic in the near future [82, 83].
5. CARBON AND NITROGEN RESOURCES
Carbon and nitrogen are the most important nutrients for C. necator. Acquiring the nutrients needs to be preferably done in a sustainable manner, which can be a challenge. In this section, different techniques to capture carbon and nitrogen from the climate, industry, or by artificial synthesis are discussed.
Carbon capture storage and utilization is the technology used to capture CO2 from industry or from the atmosphere, which can be used as a resource for the HOB [84].
Capturing CO2 from industry can be done in three ways: by pre-, post-, and oxygen-enriched combustion [84-86]. Pre combustion means that the carbon is extracted before the combustion, post combustion is captured after combustion and oxy-enriched combustion means capturing the carbon dioxide while turning the fuel into mainly hydrogen gas, which is later used as a fuel for the main process. In terms of efficiency, the preferred method depends on the system to which it is applied. For example, one would prefer applying pre-combustion capture in an integrated gasification combined cycle but oxy-combustion for pulverized coal combustion [87]. This technique always must be applied to a factory or power plant where high amounts of CO2 emissions are present.
However, the bioreactor cannot always be built close to a factory or power plant. Carbon capture from ambient air can be a solution for this. Despite the more dilute stream, the system is still faster in CO2 sequestration than the natural carbon cycle [88]. There are a few emerging technologies that can bind carbon dioxide at relatively low energy costs. Carbon capture using a guanidine sorbent captures CO2 by crystallizing, and CO2 can be released by heating the crystal at 80 to 120 degrees Celsius [89]. There are also other new emerging technologies in binding CO2 from ambient air [90, 91]. However, these methods are not suitable yet for large-scale industrial applications.
Another option to capture carbon dioxide for HOB growth is using methanogens. These bacteria produce methane and CO2 from manure and co-substrates. This reduces the input for the hydrogen oxidizing bacteria to only H2 and O2, as nitrogen is also supplied by these bacteria. However, under current hydrogen rates, this is not yet a profitable process [72].
Nitrogen is the second important resource for bacteria. The most efficient source of nitrogen is NH3 [19]. The ammonia production is usually done via the commonly commercially available Haber-Bosch process [92]. However, the production of ammonia is energy intensive: global ammonia production has an energy demand of about 1% of the global energy demand and produces 0.93% of the total greenhouse gas emissions [93].
Ammonia recovery from wastes using electrolysis and stripping is also possible but has an even higher energy demand than the Haber-Bosch process [19, 94]. The benefit of this process is that the nitrogen that is in wastewater is removed to prevent it from getting into the natural system. Hence, it is more sustainable to recover the nitrogen that is in the wastewater and use it in agriculture again [19, 20]. This might be a good sustainable option, however, the costs of 6 EUR/kg N would increase the cost of the product significantly [20].
In a recent study, a novel technique to recover nitrogen from wastewater was researched in combination with HOB growth [95]. This technique achieved up to 90% ammonia recovery from wastewater, with a maximum cost of €2,18 per kg. The first quality tests of the nitrogen showed that the nitrogen is safe to use, but it has to be very pure to use for food.
As stated in ‘Aerobic Hydrogen Oxidizing Bacteria as Single Cell Protein Source’, there are some types of HOB that use N2 as a nitrogen source. However, these are less efficient than the ones used in the Haber-Bosch process for producing NH3 [24]. This bacterium (Xanthobacter autotrophicus) has a protein content of 62% ± 6.3% and is, therefore, an interesting species to further investigate because then there is no need NH3 input.
6. APPLICATION & COMPETITOR ANALYSIS
The application of HOB protein can be compared to the application of the concentrated powder form of soy and whey protein. Soy protein is currently the most used plant-based protein for human consumption [17]. Soy concentrate is used in bars, cereals, and yogurts and is a close competitor of HOB as a non-animal-based protein source. The animal-originating protein competitor whey is a by-product of cheese production and is used in beef, dairy, bakery, confectionery and as a supplement for athletes [17]. HOB could also be used as a protein rich ingredient in many types of food and meals like Solein suggests (e.g. burgers, pastas, bread, meat substitutes, smoothies) [96]. A recent study investigated HOB as an alternative to pre-packaged food in space and concluded that consumption of hydrogen-consuming SCP was 3 times cheaper than pre-packaged foods and 5 times cheaper than artificial light algae food [97]. To examine if HOB could be a suitable and profitable innovative protein source, a qualitative and quantitative analysis is made.
6.1. Qualitative Protein Analysis: HOB, Soy and Whey
When looking at the potential of HOB, the quality and availability of its protein determine the potential use and value. The amino acid (AA) composition of C. necator happens to be slightly superior compared to other bacterial proteins and is nearly equal to the milk protein casein, which is another frequently used protein [29].
6.2. Amino Acid Composition
All essential amino acids (histidine, isoleucine, leucine, lysine, methionine, phenylalanine, threonine, tryptophan and valine) are present in C. necator (Fig. 3), which means it suffices as a singular protein source in one's diet. To cover the daily essential amino acid requirements of an average adult (62 kg) 72-96 g is required, depending on the growing medium of C. necator [98]. 121 g of soybean is needed to accomplish the same amino acid concentrations [99]. Overall, C. necator is comparable with soy and whey protein concentrates and could therefore be a good protein alternative based on its AA composition. The study of Ritala et al. [22] comparing SCPs from algae, fungi and bacteria agrees that C. necator has a similar or even better AA composition than soy and algae. Other HOB were investigated by Volova & Barashkov [34], who compared the AA profile of Alcaligenes eutrophus Z1 and Ralstonia eutropha B5786 to that of yeast, microalgae and casein, and concluded their protein profiles were similar. Thereby the protein content of these bacteria was 70% d/w, which was much higher than the 50 and 15% in yeast and wheat grain.
To describe the small differences in the AA-composition, HOB have a relatively high level of alanine and low amount of cysteine. The levels of alanine, glycine, methionine, tyrosine and valine of C. necator exceed the levels of both soy protein isolate and whey protein isolate. For aspartic acid, glumatic acid, histidine, isoleucine, lysine, proline and serine levels C. necator scores under the levels of its competitors. Even though the lysine level of C. necator is the lowest in relation to the others, Calloway & Kumar [14] suggested C. necator as an excellent supplement to cereal grains.
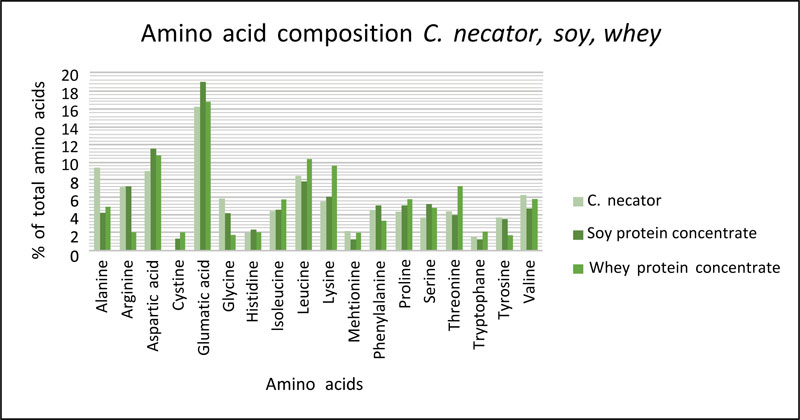
6.3. Bioavailability
The bioavailability of HOB, soy and whey proteins are displayed in Table 1. Nitrogen digestibility is the percentage of the absorbed nitrogen of the total nitrogen intake [14]. The biological value is based on the amount of nitrogen used for tissue formation divided by the nitrogen absorbed from food and multiplied by 100, expressed as percentage of nitrogen utilized [17]. The biological value indicates how efficiently the nitrogen is used by the body. The net nitrogen utilization is calculated by multiplying nitrogen digestibility by the biological value and then dividing by 100 [14]. These values were given for soy protein and whey protein [17]. The nitrogen digestibility values of soy and whey proteins, however, were calculated with the above-mentioned formula. As there is a difference in metabolism and natural diet between rats and humans, it should be taken into account that the study of Calloway & Kumar [14] used rats to test the bioavailability, whereas soy’s and whey’s values were obtained by humans.
The nitrogen digestibility of C. necator is significantly higher than that of soy and whey protein. Looking at the biological values and net nitrogen utilization, C. necator scores higher than soy, but lower than whey. These values suggest that C. necator is a better protein alternative than soy.
Protein Source | Nitrogen Digestibility % | Biological Value | Net Nitrogen Utilization % |
---|---|---|---|
C. necator boiled | 93.8 | 77.6 | 72.8 |
C. necator sonically broken | 93.3 | 77 | 71.8 |
Soy protein | 82.4 | 74 | 61 |
Whey protein | 88.5 | 104 | 92 |
6.4. Quantitative Protein Analysis
From an economical perspective, the production costs of hydrogen oxidizing bacteria should not be higher than already existing protein sources. This is already true in the case of HOB competing with space food [97]. However, when using HOB-protein as a supplement, soy and whey concentrate are considered competitors. 71-75% of C. necator’s dry weight consists of protein, which is respectively around 63% and 90% for soy and whey concentrate [14, 20]. Prices of soy protein are around 1 Euro/kg as competing for plant protein and 2 Euro/kg for the high-quality fish protein [72]. In a study by Zhang et al. [100], it was found that, with electricity costs at V0.05 kW h-1, growing a HOB culture was feasible. However, this study used a mixed culture of HOB, producing several valuable bioproducts, among which proteins. Also, no exact costs or market prices were mentioned.
6.5. Environmental Impact and Climate Resilience
The growth of HOB uses minor land occupation and water compared to the conventional ways of growing proteins such as soybeans, especially when the wind is used as an energy source [101]. It proves to be no threat to either biodiversity or the surrounding ecosystem since there is no chance of eutrophication, soil water pollution and extensive water usage. Furthermore, the production of the hydrogen oxidizing bacteria does not cause an increase in the emission of CO2, methane, or nitrous oxide, as there is no net emission of these gasses [20]. This means that for the growth in the bioreactor the carbon cycle is conserved, and there is zero additional carbon dioxide emission in the production of the product [102]. In addition, there is no fluctuation in price or yield due to seasonality, ensuring food security and stable pricing. Therefore, HOB manufacturing can provide reliable production close to the point of consumption.
6.6. Competitors and Challenges
Given the previously discussed topics, C. necator protein can compete qualitatively and quantitatively with soy protein and whey protein amongst some other SCP like fungi and algae. The technology needed to overcome the gas issues to cultivate HOB, turning it into a protein-rich ingredient, is available. There is more investigation needed on the upscaling process of the production, but this is not the limiting factor as technologies are making progress [26, 103].
Regarding food safety, especially RNA content, endotoxins, and potential allergy symptoms should be taken into account [22]. When the bacteria are grown too fast, or their RNA is not processed, the high nucleic acid content resulting in uric acid will be toxic in large quantities (>2 gram per day) [12, 23, 104]. There are several methods developed to reduce the RNA content for human consumption [12]. For example, RNAase could be used at a temperature to 60-70 °C for 20 min, alkaline hydrolysis, autolytic methods and chemical extraction. The production process of the final product of HOB-based SCP should be done carefully. Gram-negative bacteria like C. necator appear to contain endotoxins integrated in the cell walls. Endotoxins could cause inflammatory reactions [18, 105]. To inactivate endotoxins, hydrogen peroxide is used for biosynthesis of poly(3-hydroxybutyrate-co-4-hydroxybutyrate) produced by C. necator [106]. However, it is unclear how these treatments affect the quality of the proteins in HOB. An alternative method to inhibit endotoxin formation would be suppressing or the genes responsible for the toxins [18]. Allergic reactions could be minimized by destroying the cell wall using either mechanical techniques (soniciation, blending, agitation with beads, etc.) or enzymatic and chemical treatments [15, 107]. The specific details of the treatments are dependent on the species or strain and the quantity of required protein.
As HOB-protein is not yet on the EU market, it is likely to be considered as a novel food. However, before being considered novel foods, the HOB-protein product must go through an authorization and consultation process concerning implementing acts according to the European Food Safety Authority's (EFSA) regulations [108]. When confirmed as novel food by the recipient EU country, the Commission will publish information on the Commission’s website meaning that the novel food can be lawfully placed on the EU market [109]. The conditions for microorganisms as novel food are the identification of species, sufficient information for characterisation (genetic typing) at strain level by internationally accepted molecular methods, and naming of strains according to the International Code of Nomenclature [108]. In other words, HOB-protein must be produced from a pure culture. Unlike a mixed culture, which is more efficient and cheaper to produce, a pure culture is commercially unattractive as it is not cheap enough to compete with soy [110]. The need for sterilisation, and lack of symbiosis between strains make pure culturing a costly process. As long as the European Union does not adjust its regulations, this will be the bottleneck for successful implementation of HOB-protein in Europe. Consequently, countries where the regulations are less strict (e.g. US, UK, China), might succeed sooner.
HOB is produced at a large scale for bioplastics, feed (for fish and livestock) and bacterial oils [25]. To give an image of the efficiency of production of HOB for bioplastics (polyhydroxylalkanoates). A cell concentration of 150-160 kg/m^3 over a course of 60 hours in a pilot plant of 150L was obtained [111]. Some companies producing HOB for bioplastic or feed are Kiverdi (plastics) company from 2011 and Novonutrients in cooperation with Deep Branch Biotechnology (feed) [25]. Since 2019, Kivirdi also focused on producing HOB to be the first in producing meat based on air. Their product is called air protein, which is a protein powder ingredient [112]. Novonutrients started around that same year with HOB production for human consumption. Other promising companies producing HOB-based protein as food are the Finnish Solar Foods company and the Belgian Avecom company. The Belgian company Avecom collaborated with the Dutch KWR for the Power to Protein project. In the project they investigated the use HOB for food [113]. They have run a pilot bioreactor (580L) in which they produced biomass with 49% SCP and 85% digestibility (in vitro) [95]. Lab scale values were not reached, but it is a promising start. Solar foods were founded in 2017 and produce Solein as protein ingredient for human consumption [96]. In 2020 it raised funding of almost 25 million euros for ‘producing a unique single-cell protein out of thin air’ project [114]. This is a promising start for implementation of HOB-protein as ingredient on a large scale.
7. ACCEPTANCE
When it comes to consumer acceptance, there has been no research published concerning the specifics of acceptance of hydrogen-oxidizing bacteria for human consumption. Therefore, consumer acceptance of similar innovative protein products such as insects is used to review the acceptance of HOB. There are different ways to approach the acceptance of novel products. A lot of research is focused on marketing or psychology, and therefore on individuals but it is also possible to focus on societal structures [115]. When focussing on individual consumer heuristics, psychological and cultural factors are of importance [116]. When looking at structural issues, it is important to take the social and practical context into consideration and with that, the characteristics of the product [115]. A general overview of the factors that influence consumers' acceptance is given in Fig. (4).
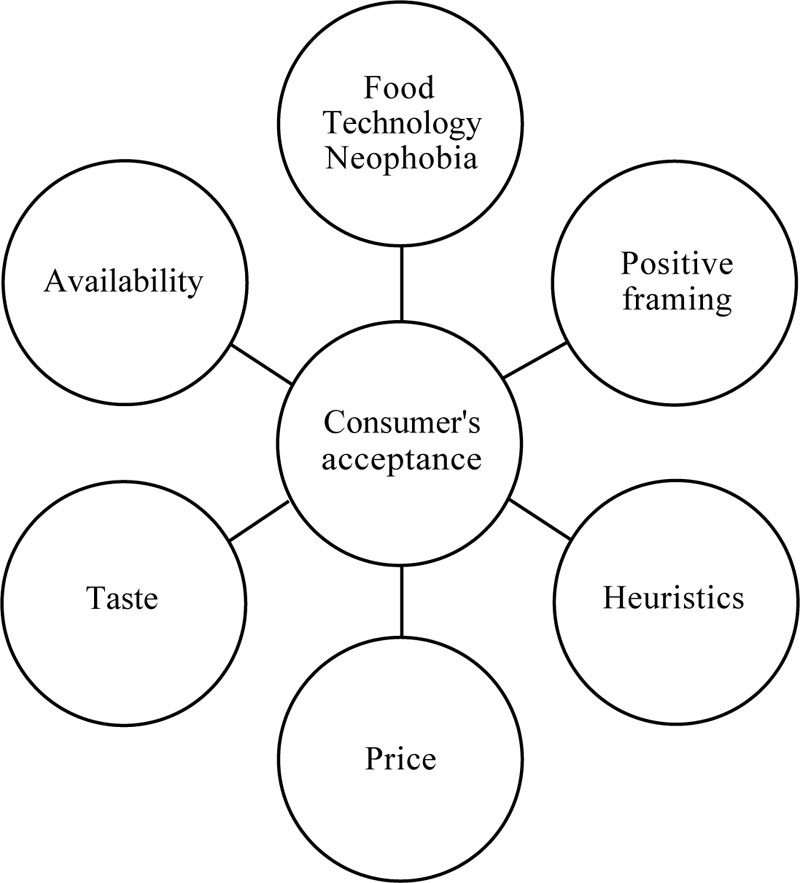
7.1. Individualist Perspective
Heuristics are important in people's willingness to accept products. The association and emotions linked to the type of product can play a crucial role. Naturalness and associations with healthiness of a product can increase positive association. Trust is also an essential heuristic. For acceptance of the idea of shared values is necessary, the consumer needs to believe the producers have the same ideas regarding the goal of the products as they have themselves [116].
What can help build a more positive association lies within the framing of the product. This can be seen in the framing of cultured meat. The way in which it is described affects the willingness to accept [117]. An example of positive framing is by using labels. Labels for organic products from an independent accredited institute can be used for differentiation by brands. Positive perception of brands due to organic labelling increases on global, local and private scale and consumers are more willing to pay a premium price for the brand [118].
The psychological factors that could play are role are food technological neophobia. Food technology neophobia refers to the willingness of people to eat novel technological products. There is a scale which can measure this [119] and it can be used in research on the acceptance of products. For example, with the production of 3D printed food, food technology neophobia is high and hard to overcome [120]. For hydro-oxidizing bacteria, further research is needed.
7.2. Structuralist Perspective
However, coming from a structuralist perspective, House [115] argues that acceptance should not be focused on getting people to try the product but on incorporating the product into their daily lives. Though characteristics such as sustainability could persuade people to buy a product once, other structural factors such as price, taste and availability are much more important in getting people to buy it more often. Furthermore, House [115] states that in the initial stage of production, the focus should be on “early adopters” who are eager to try new products rather than the average consumers who will likely follow later.
The above-mentioned theories on acceptance of similar novel food products can be used when thinking about the acceptance of HOB. However, it must be noted that every situation is different, and what can be used for one product may not work for another. Looking at the context is always important, and these theoretical frameworks can be used as tools to apply to the specific case of hydrogen-oxidizing bacteria.
8. FURTHER RESEARCH
The use of hydrogen-oxidizing bacteria as an alternative protein source has so far merely shown a fraction of its full potential and requires a vast amount of further research before a large-scale implementation is feasible. During the review assembly, several important factors were encountered for which the available knowledge proved insufficient.
Regarding the use of a certain HOB, there is relatively little published information available. Currently, C. necator has been studied most intensively and seems well applicable. However, due to lack of research other HOBs with good potential are possibly left out of the picture. Further research might lead to finding a bacterium with a higher efficiency and yield.
Additionally, the sustainable collection of nitrogen poses a challenge that is yet to overcome. The use of N2 as nitrogen source decreases the biomass yield, while the use of valuable NH3 significantly increases the price of the final product. It still has to be investigated whether NH3 can be collected in a less expensive manner or if the yield of HOB on N2 application can be increased.
Besides, little is known about the possibilities of reusing the gas flow of oxygen and hydrogen going out of the tank. A certain ratio of oxygen and hydrogen gas is led into the tank to ensure optimal growth, which is a significantly larger amount of hydrogen gas than the bacteria can ever consume. It has to be determined whether the recycling of gas is worth the effort energy-wise.
When all technical limitations have been overcome, effort should be invested in the acceptance of HOB into the general market and the food safety of the product. Extensive research should be performed to make sure the introduction of HOB goes smoothly, as a wrong first impression can hardly be undone.
In conclusion, various factors still require attention to optimize the potential of hydrogen oxidizing bacteria as a protein replacement in the food and feed industry. The current production process, choice of bacterium, experimental conditions and reactor is largely based upon research with the same means but a different goal. It would be beneficial for large-scale implementation if all aspects of reaching an optimal protein yield were investigated for HOB specifically in order to remove the hypothetical factor.
CONCLUSION
With a growing world population and the earth’ boundaries at the edge of crossing, innovative protein sources will play a crucial role in sustaining our future livelihood. In this review, hydrogen oxidizing bacteria have been presented as a sustainable option for human protein consumption from multiple disciplines. As best-described HOB, C. necator has been used as an example for this form of SCP. With the limited resources needed for cultivation, high protein content, good bioavailability and the fortunate amino acid composition compared to its competitors, HOB qualifies as a valid alternative protein source for human consumption. Implementation into the market is realistic, as other alternative protein sources (algae, insects) are already commonly used as a food supplement. Future improvements in the production efficiency of pure cultures of HOB and closely related stagnations in production costs will determine the feasibility. This will, together with the legislation and consumer acceptance, determine if this novel protein source can help to fill the global needs.
LIST OF ABBREVIATIONS
SCP | = Single Cell Protein |
HOB | = Hydrogen-oxidizing Bacteria |
(MECs) | = Microbial Electrolysis cells |
CONSENT FOR PUBLICATION
Not applicable.
FUNDING
The authors are employed at Wageningen University & Research or are otherwise directly connected to Wageningen University & Research, who funded the publishing of the present paper. The authors received no financial support for the research, authorship, and/or publication of this article from other sources.
CONFLICT OF INTEREST
The authors declare no conflict of interest, financial or otherwise.
ACKNOWLEDGEMENTS
Declared none.