All published articles of this journal are available on ScienceDirect.
Neutralizing epitope of the Fusion Protein of Respiratory Syncytial Virus Embedded in the HA Molecule of LAIV Virus is not Sufficient to Prevent RS Virus Pulmonary Replication but Ameliorates Lung Pathology following RSV Infection in Mice
Abstract
Aims:
To develop experimental bivalent vaccines against influenza and RSV using a cold-adapted LAIV backbone.
Background:
Respiratory syncytial virus (RSV) is a causative agent of bronchiolitis and pneumonia in young children, elderly and immunocompromised adults. No vaccine against RSV has been licensed to date for various reasons. One of the promising platforms for designing RSV vaccine is the use of live attenuated influenza vaccine (LAIV) viruses to deliver RSV epitopes to the respiratory mucosa.
Objective:
To generate recombinant LAIV viruses encoding a neutralizing epitope of the RSV fusion protein and assess their protective potential against both influenza and RSV infections in a mouse model.
Methods:
Reverse genetics methods were used to rescue recombinant LAIV+HA/RSV viruses expressing chimeric hemagglutinins encoding the RSV-F epitope at its N-terminus using two different flexible linkers. BALB/c mice were intranasally immunized with two doses of the recombinant viruses and then challenged with the influenza virus or RSV. The LAIV viral vector and formalin-inactivated RSV (FI-RSV) were included as control vaccines. Protection was assessed by the reduction of virus pulmonary titers. In addition, RSV-induced lung pathology was evaluated by histopathology studies.
Results:
Two rescued chimeric LAIV+HA/RSV viruses were identical to the LAIV vector in terms of replication capacity in vitro and in vivo. The RSV-F neutralizing epitope was successfully expressed only if inserted into the HA molecule via G-linker, but not A-linker. Both chimeric viruses induced high influenza-specific antibody levels and fully protected mice against a lethal influenza challenge virus. However, they induced weak anti-RSV antibody responses which did not prevent RS virus replication upon challenge, and only LAIV-HA+G-RSV variant protected mice against RSV-induced lung pathology.
Conclusion:
Although the designed LAIV-RSV chimeric viruses were unable to neutralize the RS virus pulmonary replication, the LAIV-HA+G-RSV reduced RSV-induced lung pathology and can be considered a promising bivalent vaccine against influenza and RSV infections and warrants its further development.
1. INTRODUCTION
Human Respiratory Syncytial Virus (RSV) is a causative agent of bronchiolitis and pneumonia responsible for fatalities among children under 5 years old and elderly worldwide [1]. Since vaccination is the main strategy in the fight against infectious diseases, there are ongoing efforts to develop safe and effective RSV vaccines. Despite this, all RSV vaccine candidates tested to date have failed to progress beyond Phase 2 or failed to meet their primary endpoints in Phase 3 [2]. The main reason vaccines have not been available for decades is limited knowledge of the complex interactions between the virus and the host, especially in humans. Furthermore, the development of RSV vaccine has been challenging due to the failure of the alum-adjuvanted formalin-inactivated RSV (FI-RSV) vaccine studied in infants and toddlers in the 1960s, which resulted in Enhanced Respiratory Disease (ERD) after subsequent exposure to a natural RSV infection: the majority of the vaccinees required hospitalization and two vaccinated children died [3, 4]. All potential RSV vaccines are being designed so as not to cause these ERD conditions, but such vaccine candidates cannot be generated by classical vaccine development methods, and new innovative strategies for RSV vaccine development are of high priority.
One of the strategies involves the insertion of an RSV protective antigen into another virus (called a “vector”) that delivers selected RSV epitopes into an organism and thus induces protective immunity against RSV. Two surface glycoproteins F and G are the main targets for neutralizing antibodies, and are most often used for vaccine development. The attachment RSV G protein is known to enhance pathogenicity in vivo and mediate the attachment to the host cell [5]. The G protein is considered one of the most variable RSV proteins due to its structure, as it consists of disordered, highly glycosylated mucin-like domains, which complicates its use in the development of viral-vectored vaccines [6-8]. Nevertheless, some of the G-directed human antibodies are sufficient to protect mice from RSV. For example, 3D3 and 3G12 antibodies binding to the central conserved region of RSV-G facilitate the neutralizing activity directed to both RSV strains [9, 10]. The RSV F protein is responsible for the fusion process between the host and the viral membrane. The virus penetrates into host cells by transforming the metastable conformation before fusion (pre-F) into a highly stable conformation after fusion (post-F) [11, 12]. These two RSV proteins are widely used for designing vectored vaccines based on such viral vector platforms as modified vaccinia Ankara virus [13], human parainfluenza virus type 3 [14], human or chimpanzee adenoviruses [15, 16], or vesicular stomatitis virus (VSV) [17].
Attenuated influenza viruses are considered an attractive vector for the delivery of RSV epitopes to the respiratory tract, mainly due to the known ability of influenza viruses to induce all arms of adaptive immune responses, and also because such vaccines can be used for combined protection against the two most dangerous human respiratory pathogens [18]. Unlike other viral vectors, influenza viruses have limited genome capacity for the insertion of foreign antigens, therefore influenza virus vectored vaccines are designed to carry selected B- or T-cell epitopes. For example, the RSV-F antigenic site II is recognized as a Palivizumab-binding site and is conserved among both RSV A and B strains and on both conformations of F protein (pre-F and post-F). According to previously reported results, a vaccine candidate based on the A/PR/8/34 (H1N1) [PR8] virus, which encoded the neutralizing epitope into the N-terminus of the hemagglutinin HA1 subunit was shown to be protective against RSV A2 without causing the vaccine-enhanced disease [19]. However, the use of the PR8 virus backbone for live viral-vectored RSV vaccine is not clinically relevant due to safety concerns. In the current study, we generated recombinant influenza viruses carrying the RSV-F neutralizing epitope on a cold-adapted influenza virus backbone widely used as live attenuated influenza vaccine (LAIV) and studied these candidates for their protective potential against both influenza and RSV infections in a mouse model.
2. MATERIALS AND METHODS
2.1. Cells, viruses, plasmids, antibodies
2.1.1. Cells
The MDCK and Hep-2 cell lines were grown in Dulbecco's Modified Eagle's Medium (DMEM) (Gibco, USA) containing 10% heat-inactivated fetal bovine serum (FBS) (Capricorn Scientific, Germany) and antibiotic-antimycotic solution (AA) (Thermo, USA). The original LAIV and LAIV-RSV vaccine candidates were rescued by transfecting Vero cells that have been adapted to grow in serum-free OptiPRO medium (Thermo, USA). All cell cultures were grown at 37°C in a humidified incubator containing 5% CO2.
2.1.2. Viruses
An LAIV virus used as a viral vector in this study was rescued previously and contained hemagglutinin (HA) and neuraminidase (NA) from the A/Anhui/1/2013 (H7N9) virus and six remaining gene segments from the A/Leningrad/134/17/57 (H2N2) [Len/17] master donor virus [20]. A virulent influenza virus A/Shanghai/2/2013(H7N9)-PR8-IDCDC-RG32A [H7N9-PR8] comprising of HA and NA genes from the A/Shanghai/2/2013 (H7N9) virus and the remaining genes of the PR8 virus was obtained from the Centers for Disease Control and Prevention (CDC, Atlanta, USA). Influenza viruses were propagated in 10-11-day-old embryonated chicken eggs at 33°C (for Leningrad-based vaccine viruses) or at 37°C (for the PR8-based virus) for 48 hours. Harvested allantoic fluid was clarified by low-speed centrifugation and supernatants were stored in single-use aliquots at -70°C. Virus titers were determined by standard end-point titration in eggs and expressed as lgEID50/ml.
RSV A2 strain was obtained from the Smorodintsev Research Institute of Influenza (Saint Petersburg, Russia). RS viral stock was produced in Hep-2 culture and prepared as described previously [21]. Briefly, the Hep-2 fully confluent monolayer was infected with ten-fold diluted RSV A2 in DMEM+AA at 37°C. Two hours later, the DMEM+AA containing 1% FBS was added and cells were incubated at 37°C for five days. The RSV stock was harvested as a cell-free media after low-speed centrifugation, aliquoted and frozen at -70°C until use. RSV A2 titers were measured as described previously [21] and expressed in plaque-forming units (PFU) per ml. The formalin inactivated vaccine (FI-RSV) preparation was made by mixing formalin (Sigma Aldrich, USA) and RSV stock to a ratio of 1:4000 and incubation at 4°C for 72 hours. The treated virus was centrifuged at 30,000 g for 1 h and resuspended in Dulbecco's Phosphate-Buffered Saline (DPBS) (Thermo, USA).
2.1.3. Plasmids
An RSV-F fragment (residues 243-294) was chemically synthesized and cloned into pAL2-T vector by Evrogen Ltd. (Moscow, Russia). A set of eight dual-promoter pCIPolISapIT plasmids encoding all genes of Len/17 master donor virus were generated prior to the commencement of the study [22].
2.2. Rescue of Recombinant Influenza Viruses
The RSV-F243-294 fragment was cloned between the signal peptide and the HA1 subunit of the H7N9 HA molecule using either AAAPGAA or (GGGGS)2 linker (Fig. 1A). The LAIV-RSV viruses were rescued by electroporating Vero cells with a set of eight plasmids encoding all influenza genes using the Neon 100 transfection system (Thermo, USA). Three days after transfection, the mixture of transfected media and cells was injected into eggs, which were further incubated at 33°C for 48 hours.
2.3. 3D Modelling
The spatial structure of the RSV-F243-294 fragment with A-linker or G-linker was simulated ab initio using the I-TASSER algorithm [23]. The I-TASSER algorithm calculates protein folding based on the assumed similarity of individual fragments of the known structures from the protein database (PDB) libraries, with the subsequent choice of the optimal variant of folding based on the energy of the resulting folded molecule. To verify the constructed model, the assembly of fragments is simulated, followed by the selection of the optimal model.
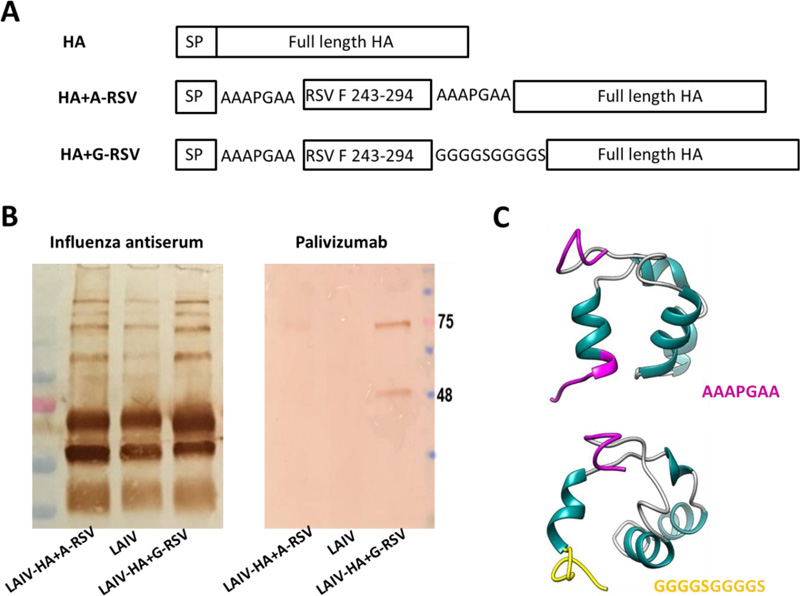
2.4. Viral Growth In Vitro
Growth characteristics of rescued LAIV-RSV viruses were assessed in eggs and MDCK cells. Viral titers were compared in eggs at different temperatures to determine the temperature-sensitive and cold-adapted phenotypes of the recombinant viruses, as previously described [24]. In addition, viral replication on MDCK cells was assessed. In brief, the LAIV-RSV candidates were serially diluted in DMEM+AA containing TPCK-trypsin (Sigma-Aldrich, USA) and 25 µl of the diluted virus was inoculated into each well of confluent 96-well plates for 1 hour at 33°C. Then the viral inoculum was removed and DMEM+AA containing TPCK-trypsin was added, followed by incubation at 33°C for 3 days. The presence of the virus was determined by hemagglutination assay and viral titers were determined using the Reed and Muench method and expressed as lgTCID50/ml.
2.5. Western Blot Analysis
Sucrose gradient-purified LAIV or LAIV-RSV were used in WB analyses. Protein concentrations of the purified influenza viruses determined using the Pierce BCA Protein Assay K (Thermo Fisher Scientific, USA). To detect influenza virus antigens, a polyclonal mouse antiserum was produced after intranasal infection of BALB/c mice with two sub-lethal doses of H7N9-PR8 virus 14 days apart.
The purified LAIV-RSV and LAIV in 2 repeats (sets) were separated by SDS-PAGE using pre-cast 12% PAAG cassettes (Bio-Rad, USA), followed by the transfer of the protein bands onto nitrocellulose membrane (Bio-Rad, USA). After washing with PBS containing 0.05% Tween 20 and blocking with 5% skim milk, the membrane was incubated with either sera from mice infected with H7N9-PR8, or with Palivizumab monoclonal antibody for 2 hours. Then the first membrane was labeled with horse-radish peroxidase (HRP)-conjugated goat anti-mouse IgG (Sigma, USA), and the second one with HRP-conjugated goat anti-human IgG (Sigma, USA. The protein bands were developed using DAB substrate (Vector Laboratories, USA).
2.6. Mouse Studies
All mice experiments were approved by the Local Ethics Committee of the Institute of Experimental Medicine, Saint Petersburg (Approval Nº3/19 from 25.04.2019). Six to eight weeks-old female BALB/c mice were supplied by Stolbovaya laboratory animal breeding nursery (Moscow region, Russia). All efforts were made to minimize mice suffering; thereby immunization, infection and bleeding via orbital puncture were performed under light anesthesia with ether.
2.7. Immunization Schedule and Challenge
Three groups of 13 mice were inoculated intranasally (i.n.) with two doses of 1×106 EID50 of LAIV, LAIV-HA+A-RSV or LAIV-HA+G-RSV. in a volume 50 µl, three weeks apart. The control mice (n=9) received i.n. PBS at the same time points. An additional group of 5 mice received two intramuscular injections of 2 μg of FI-RSV absorbed to AlumVax Hydroxid (Oz Biosciences, France), with a 2 week interval. On the third day after the first i.n. vaccine administration, the influenza viral titers in nasal turbinates and lungs were measured for 4 mice in each group. Briefly, the collected organs were homogenized in 1 ml of DPBS supplemented with AA in TissueLyser LT (QIAGEN, Germany) small bead mill, followed by the removal of tissue components by low-speed centrifugation. Then chick embryos were inoculated with 100 µl of serially diluted homogenates to yield the EID50 values. Blood samples were drawn three weeks after the second immunization and centrifuged at 3000 rpm for 6 min to obtain sera for immunogenicity analyses.
Immunized mice were divided into two groups for challenge experiments. The first group (n=4) was infected i.n. with 1×105 EID50 of H7N9-PR8 influenza virus in a volume 50 ul. The lungs and nasal turbinates tissues were collected three days post influenza challenge to determine influenza viral titer as described above. The remaining mice, including animals treated with FI-RSV (n=5 per group), were infected with 5×105 PFU of RSV A2, which was administered dropwise to the nose. Five days later, the whole lungs were removed for examination. For histology analysis, one lung lobe (superior lobe of the right lung) was immersed into 10% formaldehyde for a minimum of 12 hours. The remaining lung samples were prepared in the same manner (as described before) to perform the plaque assay and to calculate corresponding RSV titer in plaque-forming units per gram tissue, as described previously [21].
2.8. Assessment of Immune Responses
Anti-H7N9 and anti-RSV antibodies were measured by standard ELISA, as described previously [25]. ELISA microplates (Corning, USA) were coated with 50 ng of sucrose gradient-purified H7N9-LAIV virus or 200 ng of purified FI-RSV at 4°C overnight. The non-specific binding was blocked using 5% skim milk. The 2-fold diluted mice sera were added to the plate as a primary antibody, followed by incubation with HRP-conjugated anti-mouse IgG (Sigma, USA). The colorimetric reaction was developed in plates using 3,3′,5,5′-Tetramethylbenzidine (TMB, Thermo Fisher Scientific, USA) substrate, then stopped with 2M sulfuric acid and the optical density (OD) was measured at 450 nm. The level of anti-H7N9 antibody was determined by calculating the area under the curve (AUC) of OD450 values using the trapezoidal rule. The levels of anti-RSV antibody were generally low and were presented as the mean±SEM of the OD450 value of the serum dilution 1:10.
2.9. Histology Study
Tests of the bronchopneumonia factors that accompany the RSV infection, including the induction of pulmonary eosinophilia were carried out in the main regions of the upper lung lobes. The study was conducted as described by Albert et al. [26]. The paraformaldehyde-fixed lungs were embedded in paraffin and cut into sections of 5 μm thickness. The lung sections were stained in a 1% ethanolic solution of Congo Red (Sigma), counterstained in Karazzi's haematoxylin for 10 min, followed by 10 min incubation with Alcian Blue 8GX stain at pH 2.5 for mucus detection in bronchial lumen. Four to five sections per lung were scored for the degree of epithelial damage, peribronchovascular cell infiltrate, and interstitial-alveolar cell infiltrate. For each histology section, a blinded histologist assigned a value of 0 to 3 depending on the degree of epithelial damage or inflammation.
3. RESULTS
3.1. Generation and in vitro Characterization of Recombinant LAIV+HA/RSV Vaccine Candidates
Chimeric LAIV+HA/RSV vaccine viruses were rescued in Vero cells and amplified in eggs. The two recombinant viruses differed only by the flexible linker between the RSV-F243-294 and the HA1 subunit of influenza H7 hemagglutinin (Fig. 1A). The vaccine candidates were named LAIV-HA+A-RSV (AAAPGAA linker) and LAIV-HA+G-RSV (GGGGSGGGGS linker). Both viruses were fully sequenced to confirm their genetic identity and the absence of spontaneous unwanted mutations. Western Blot analysis with monoclonal antibody Palivizumab was performed to prove that the RSV-F243-294 insert is indeed expressed within the HA molecule. Surprisingly, two specific bands reacting with this antibody were detected only in the LAIV-HA+G-RSV virus and corresponded to the HA1 subunit (~55 kDa) and HA0 precursor (~75 kDa) influenza proteins, suggesting that the RSV epitope is indeed expressed along with the influenza virus HA protein (Fig. 1B). These bands were barely seen in the LAIV-HA+A-RSV group, suggesting that the linker between RSV epitope and the influenza hemagglutinin HA1 subunit plays a significant role in the chimeric protein folding. We used an i-TASSER algorithm to predict 3D structures of the RSV243-294+linker fragments. Thus, the α-helix in the region of 52-60 a.a. of RSV-F+A-linker affects the twisting of Ala residues into an ordered structure, thereby reducing the length of the flexible part of the linker, which may negatively affect the presentation of the RSV insert on the surface of the virion (Fig. 1C). In contrast, this effect was not observed for the RSV-F+G-linker construct, due to the features of Gly residue. Furthermore, the formation of a secondary structure is not observed for the AAAPGAA linker, which is present at the beginning of each of the 2 inserts and divides the RSV-F insert and the HA signal peptide (Fig. 1A, C).
Importantly, the insertion of the RSV-F fragment into HA molecule of the H7N9 LAIV virus did not affect the replicative properties of the recombinant viruses: both vaccine candidates were able to grow in eggs to high titers at the temperature optimal for LAIV viruses (33°C, Fig. 2A). In addition, both chimeric LAIVs were able to grow efficiently at 26°C, i.e. preserved the cold-adapted phenotype (the difference with 33°C did not exceed 3.0 lgEID50). Most importantly, these viruses retained the ts phenotype, i.e. poorly replicated at 38°C (Fig. 2A). We further tested replicative capacity of the rescued viruses on MDCK cells. Both recombinant LAIVs had comparable infectious titers in the cell culture as the control H7N9 LAIV virus (Fig. 2B), suggesting that the foreign insert had no effect on the LAIV virus replicative properties in vitro.
3.2. Replication of Recombinant LAIV-RSV/F Candidates in Mouse Respiratory Tract and Induction of Protective Anti-influenza Immunity
Both recombinant LAIV-RSV/F candidates were able to replicate in the upper respiratory tract of BALB/c mice after intranasal inoculation (Fig. 3A). Importantly, both chimeric viruses, as well as the LAIV vector, were attenuated for mice, since no infectious virus was isolated from the lungs of immunized mice in any of the study group (Fig. 3A). Strikingly, the insertion of the RSV-F243-294 fragment into the LAIV genome resulted in the induction of enhanced influenza-specific IgG antibody responses (Fig. 3B). These data suggest that the expression of the RSV fragment on the surface of the LAIV viral particle may result in a better presentation to the immune system of the HA protein itself, thus inducing higher levels of HA-binding IgG antibody. The induced anti-influenza antibody fully protected mice against homologous influenza virus infection: none of the vaccinated mice shed the challenge virus in the lower respiratory tracts (Fig. 3C). Therefore, the experimental recombinant vaccines remained fully functional in terms of induction anti-influenza immunity, with no negative effect seen for the inserted foreign antigen.
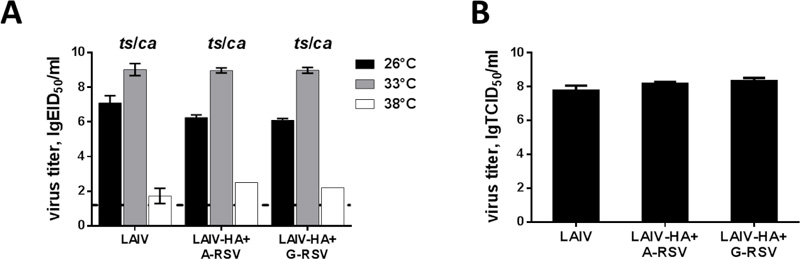
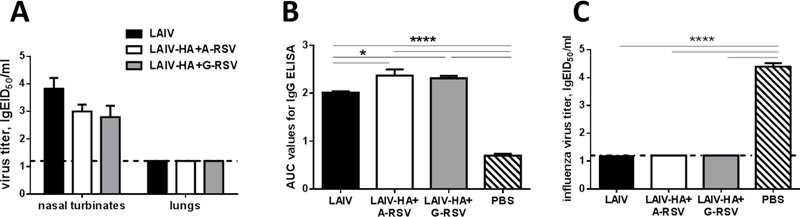
3.3. Immunogenicity and Protection Against RSV Infection and RSV-induced Pathology
Anti-RSV serum IgG antibody was measured to the whole RS virus by ELISA. Both LAIV-RSV/F viruses failed to induce detectable levels of anti-RSV antibody, whereas the FI-RSV vaccine was significantly more immunogenic (Fig. 4A). These data suggest that the whole RS virus antigen could capture serum antibody with various specificity from the FI-RSV-immunized mice, whereas the LAIV-RSV/F recombinant viruses could induce only a selected population of the antibody targeted to the antigenic site II, which is present in a very small quantities on the surface of the whole RS virus. To detect the RSV-specific antibody, an antigen corresponding to this antigenic site should be used to coat ELISA plates. As expected, FI-RSV vaccine fully neutralized RS virus replication in the lungs, whereas the LAIV-RSV/F chimeric vaccines were unable to prevent RSV infection (Fig. 4B). Although there was no significant difference seen between the two chimeric vaccines, significant reduction of the pulmonary RSV titer compared to the PBS-immunized group was observed only in the LAIV-HA+G-RSV group, suggesting that the G-linker is advantageous for correct presentation of the RSV fragment within the chimeric HA-RSV protein (Fig. 4B).
To find out if the LAIV-RSV/F viruses can protect mice against live RSV infection without causing the immunopathology, we performed the histopathological evaluation of lung tissues of immunized mice on day 5 after challenge with live RSV A2 strain. Importantly, despite full protection against RS virus replication induced by the FI-RSV vaccine, this neutralization was accompanied by severe immunopathology: mouse lungs from this group had signs of severe epithelial damage and high level of interstitial-alveolar infiltrate (Fig. 5A, B). In addition, mucus in the bronchial lumen was detected only in the FI-RSV group, as evidenced by the Alcian blue staining (Fig. 5A). In agreement with other studies, the FI-RSV vaccine developed eosinophilia, whereas neither the control group, nor the i.n. vaccine groups induced this pathology (Fig. 5B). In general, changes in pulmonary septa in the FI-RSV group were characterized by lymphohistiocytic infiltration, which is specific for viral infections. An uneven thickening of the alveolar septa due to proliferation and hypertrophy of type 2 pneumocytes with immature multilamellar bodies was noted in the FI-RSV-immunized mice. Overall, histopathological evaluation of lung sections of RSV-challenged mice revealed a high degree of protection by LAIV-RSV/F vaccines, with evidence of slightly higher protection against pulmonary pathology in the LAIV-HA+G-RSV vaccine, compared to the LAIV-HA+A-RSV group. Thus, significant protection against bronchoepithelial damage was seen between the LAIV vector and the LAIV-HA+G-RSV vaccine, but not the LAIV-HA+A-RSV candidate (Fig. 5B). These findings indicate that the incorporation of the RSV-F neutralizing epitope into the influenza HA1 subunit through the (GGGGS)2 linker could have played a role in better protection against RSV disease, compared to the AAAPGAA linker.
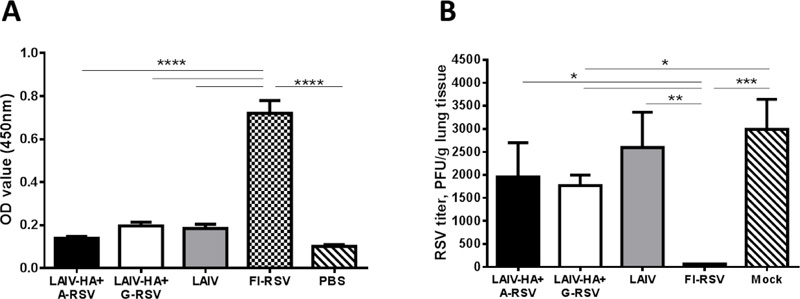
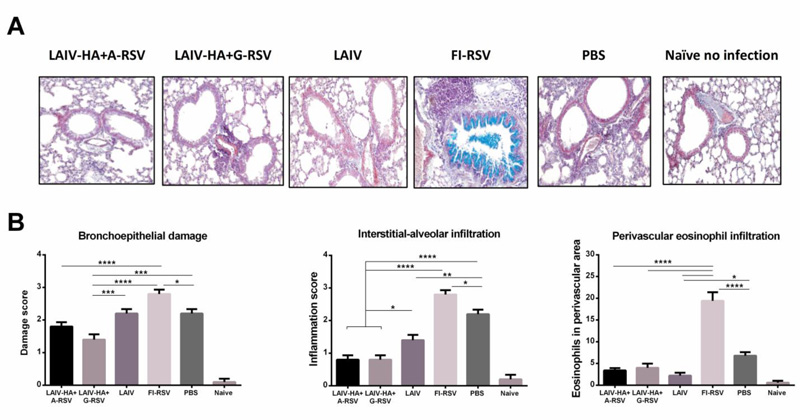
4. DISCUSSION
Since the RSV identification in the 1950’s, there have been multiple attempts to develop a vaccine to control RSV infection in humans. The RSV vaccine development hesitancy occurred after the devastating outcomes of the clinical trials of formalin-inactivated whole-RSV vaccine in the 1960s [3]. Subsequent studies identified an enhanced pulmonary inflammatory response with a predominant Th2-like cytokine pattern in mice immunized with the FI-RSV vaccine upon natural RSV infection [27, 28]. This disease enhancement effect was not seen when mice were primed with live RSV, suggesting that the formulation and the route of immunization are critical for a vaccine’s safety [27]. These data provided a rationale for the development of a live attenuated RSV vaccine, however such vaccines developed by classical cold adaptation or chemical mutagenesis have not been successful in clinical development [29]. Since then, multiple alternative approaches have been elucidated to generate a safe and effective RSV vaccine, and currently, there are many experimental RSV vaccine candidates in pre-clinical and clinical development [30, 31]. The majority of RSV vaccines under development are intended to induce neutralizing antibodies to the RSV F protein, which can be present in two distinct conformations with different content of neutralizing epitopes [32]. In our current study, we used a neutralizing epitope residing in antigenic site II of the RSV F protein, which is stable in both conformations, as a target for RSV vaccine development. The idea of inserting the F243-294 Palivizumab-targeted epitope into the HA molecule of the influenza virus was originally suggested by Lee et al. [19]. In that study, the RSV epitope was fused with the HA1 subunit of the PR8 virus using the AAAPGAA flexible linker. We used the same strategy to generate the recombinant influenza virus using an attenuated H7N9 LAIV virus as a backbone. Surprisingly, in contrast to the study by Lee et al., the expression of the RSV epitope by the recombinant LAIV virus was not detected by Western Blot analysis. Therefore, we generated an additional LAIV-RSV/F vaccine using another flexible linker. Strikingly, the G-linker afforded better spatial organization of the foreign epitope, and our 3D modelling analysis suggested the involvement of the Ala residues located in the A-linker into generation of the ordered structure of α-helix, thus limiting the independent folding of the foreign antigen. To find out if this better exposure of the RSV-F epitope on the surface of the viral particle could affect the protective properties of the vaccine, we used both constructs in further mouse experiments.
Despite the correct presentation of the inserted RSV epitope on the surface of the viral particles, this fragment was low immunogenic when delivered to the respiratory mucosa. The limitation of our study is that we used the whole RSV antigen to measure the IgG antibody in ELISA. The use of a recombinant RSV-F fragment would likely lead to higher levels of RSV-specific antibodies. Nevertheless, the RSV-targeted antibody levels induced by our vaccines were not sufficient to significantly neutralize RS virus pulmonary replication, which is also in contrast to the study by Lee et al. [19]. These discrepancies are most likely due to the distinct patterns of the vaccine virus replication in the mouse respiratory tract: our vaccines were completely attenuated and did not replicate in mouse lungs, whereas the PR8-based recombinant vaccine grew up to the titer 106 EID50 by day 6 post-infection [19]. Importantly, even with these weak anti-RSV antibody responses, our constructs protected mice from RSV-induced immunopathology, whereas FI-RSV vaccine caused exacerbated disease upon RSV infection, even though RS viral pulmonary replication was not observed in this group. It remains to be determined if the weak anti-RSV antibody induced by LAIV-RSV/F played a role in the protection, or whether there were CD8 T-cell responses induced to the RSV MHC class II epitopes located within the RSV-F243-294 fragment [33]. Although we did not measure T-cell responses in this study, in our previous experiments with LAIV-RSV chimeric vaccines expressing H2-Kd-restricted T-cell epitopes of the RSV M2-1 protein demonstrated the establishment of strong, fully functional RSV-specific CD8 T-cell responses which protected BALB/c mice against RS virus pulmonary replication and immunopathology [21, 34]. Another possible explanation of the protective effect of the LAIV-RSV/F constructs against RSV-induced lung pathology is that the productive influenza viral infection induces type I interferon (IFN) responses [35], and IFNα has been shown to non-specifically reduce RSV-induced lung inflammation [36]. However, in this case the LAIV vector control group would also protect animals against RSV-induced lung pathology.
Noteworthy, the anti-influenza immunity was not compromised by the insertion of the foreign epitope into LAIV genome, thus facilitating the development of the recombinant vaccines as bivalent vaccine preparations. In fact, the anti-influenza IgG antibody levels were significantly higher in the chimeric LAIV-RSV groups, compared to the LAIV vector control group. This phenomenon could possibly be explained by the assumption that the insertion of a foreign epitope into the viral HA molecule could result in the exposure of additional HA antigenic sites, thus inducing higher influenza-specific antibody titers. It should be emphasized that the strategy of inserting a foreign antigen into the influenza HA molecule used in our study, or into NA protein used by others [37], requires the generation of a new chimeric influenza virus gene for each influenza virus subtype, which will limit the use of such constructs as a seasonal influenza-RSV bivalent vaccines. This obstacle can be overcome by designing chimeric NS genes carrying RSV epitopes [21, 38], and this gene can be combined with any desired HA and NA genes of seasonal or pandemic influenza viruses.
CONCLUSION
The results of our study demonstrate that the designed LAIV-RSV chimeric viruses fully protected mice against lethal influenza virus infection but were unable to neutralize the RS virus pulmonary replication. However, the LAIV-HA+G-RSV chimeric virus was able to reduce RSV-induced lung pathology and can be considered a promising bivalent vaccine for the protection against influenza and RSV infections and warrants its further pre-clinical and clinical development.
ETHICS APPROVAL AND CONSENT TO PARTICIPATE
All mice experiments were approved by the Local Ethics Committee of the Institute of Experimental Medicine, Saint Petersburg, Russia (Approval No. 3/19 from 25.04.2019).
HUMAN AND ANIMAL RIGHTS
No humans were involved in this study, the reported experiments on animals were in accordance with the standards of of the Institute of Experimental Medicine, Saint Petersburg, Russia.
CONSENT FOR PUBLICATION
Not applicable.
AVAILABILITY OF DATA AND MATERIALS
The authors confirm that the data supporting the findings of this research are available within the article.
FUNDING
This work was supported by the Russian Science Foundation Grant No. 17-75-20054.
CONFLICT OF INTEREST
The authors declare no conflict of interest, financial or otherwise.
ACKNOWLEDGEMENTS
Declared none.