All published articles of this journal are available on ScienceDirect.
Microbiological Assessment and Evaluation of Virus Yield in Germ-free Chicken Eggs
Abstract
Background
Increasing yield will have major implications for vaccine manufacturers. Internal bacterial contamination of specific pathogen-free (SPF) eggs results in batches of vaccines being rejected and may negatively impact vaccine yield.
Objective
The objectives of this study were to analyze Germ-Free (GF) eggs microbiologically and evaluate the yield of H1N1 when compared with SPF eggs from 2 different suppliers.
Methods
The contents of GF eggs were analyzed using three different methods (sterility test, total viable count, and bioluminescence). Eggs from each source were inoculated with H1N1 virus. Chick Embryo Fibroblast (CEF) cell cultures were generated from GF and SPF eggs from both suppliers and infected with H1N1. The supernatant was titrated for viral Haemagglutinin activity and virus infectivity while cellular RNA extracts were assayed for Interferon (α and β) gene expression by RT-qPCR using gene-specific primers.
Results
The analysis of GF eggs confirmed that the contents were sterile. The batch yield of GF eggs was ~1.2-fold and ~1.8-fold higher than SPF supplier 1 and SPF supplier 2 virus yields, respectively. Replication of H1N1 was 2-fold higher in CEF derived from GF eggs than CEF derived from SPF supplier 1 or SPF supplier 2. IFN-β antiviral response of GF-derived CEF cells was 2-fold lower than SPF supplier 1-derived and ~3-fold lower than SPF supplier 2-derived CEF cells.
Conclusion
The content of GF eggs was sterile. The yield of H1N1 was higher in GF eggs than in SPF eggs, and the higher yield was associated with a significant reduction in interferon Beta levels.
1. INTRODUCTION
Specific pathogen-free (SPF) eggs have long been used as a substrate for vaccine production against various human and animal pathogens, such as Influenza [1-3], Yellow Fever [4], Measles and Mumps [5], Newcastle Disease [6], Smallpox [7], etc. SPF eggs are defined as eggs derived from avian flocks free from specified pathogens, as described in chapter 5.2.2 of the European Pharmacopoeia [8].
However, the use of eggs in vaccine production is not without its challenges. One of the main challenges is the risk of contamination. Microbial contamination can be a major problem, with entire vaccine batches being destroyed, delaying vaccine release and costing millions of dollars per annum. However, bacterial contamination can mainly compromise the safety and quality of vaccines [9, 10].
Despite all efforts, the risk of contamination in egg-based vaccines cannot be fully eliminated due to the unavoidable internal contamination of SPF eggs [11, 12].
The internal contamination of eggs is caused by both the unique anatomy of the avian species and the porosity of the shell [13, 14]. The pores of the eggshell are particularly vulnerable to bacterial ingress during laying and for approximately 5 minutes after the egg is laid, whilst the outer shell cuticle dries [15]. Additionally, the reproductive tract merges with the digestive tract (in the cloaca) with a single point of exit from the hen for eggs and faecal matter. This results in the porous egg coming into contact with the faeces of the chicken before being laid and consequent contamination of the fertilised egg within the shell [16]. As a result, bacteria are inevitably present on the surface of all eggs, even SPF, which then leaches in variable proportions within the egg [17, 18].
Several strategies have been developed to mitigate contamination risks in egg-based vaccine production, but the risk cannot be eliminated unless the problem of egg contamination is solved. Germ-free (GF) eggs are produced under aseptic conditions and are free from any internal microbial contamination, offering a final solution to these challenges.
Germ-Free eggs also have the potential to increase viral replication, allowing a higher viral yield in vaccine production due to the lack of gut flora in the laying hens and reduced interferon in Germ-Free eggs.
Viruses suppress or evade the innate immune response of the host as a strategy to enhance their replication, e.g., Influenza viruses block the release of type I interferon (IFN-β) through the translation of non-structural protein-1 (NS1) [19, 20], and picornaviruses evade host innate immune response through several methods [21]. Contamination of SPF eggs with non-specified pathogens may result in ‘immune-modulated’ virus yield with resultant effects on vaccine production and therapeutic applications.
Ichinohe et al. (2011) [22] showed that mice that lacked gut flora had 10-fold higher influenza viral titers than mice with functional gut flora. Abt et al. (2012) [23] also showed that the depletion of the gut flora resulted in an impaired capacity to limit viral replication due to impaired interferon responses. These results were also found in chickens, where a reduction in the interferon response during influenza infection resulted in a higher viral yield being produced [24].
Hence, the objectives of the study were to a) analyze Germ-Free eggs microbiologically for the presence of internal contamination and b) to evaluate if an increase in viral yield in Germ-Free eggs, when compared with SPF eggs, can be demonstrated due to a reduced level of interferon.
2. MATERIALS AND METHODS
2.1. Microbiological Assessment of Germ-free eggs
2.1.1. Minimal Sample Size Determination
A power calculation was performed using a simulation with R software. The calculation was performed to evaluate 80% power to reject the null hypothesis that the proportion of microbiologically contaminated eggs is greater than 0.0001% in favor of the alternative hypo- thesis that the maximum proportion is 0.0001% (one in one million).
This calculation was based on an assumed proportion of 1% or higher in the case that the null hypothesis is false, with an exact binomial test and a 5% level of significance.
A sample size of 160 eggs would be needed to demonstrate that the proportion of bacterially conta- minated eggs is, at most, 0.0001% (one in one million).
2.1.2. Microbiological Analysis by Independent Laboratory
A total of 386 eggs, produced by hens in Ovagen’s Germ Egg Production Facility, were sent to an independent specialized Contact Laboratory- Complete Laboratory Solution (CLS), Galway, Ireland, to be tested for the presence of bacteria (aerobic, anaerobic, and microaerophilic) and fungi. The eggs were sent in 17 consignment groups. For this test, the eggs were pooled to be tested according to the number of eggs delivered each time.
Each eggshell was disinfected with sterile isopropyl alcohol (IPA) 70%. The egg was then broken using a sterile spatula, and the content (white and yolk) was aseptically transferred to a sterile container. The eggs were pooled in batches of different sizes (Table 1), and 10ml of pooled eggs were transferred into Tryptone Soy Broth (TSB) in 90ml containers which was used for sample enrichment.
Each pooled sample was incubated for 24h at 30°C to 35°C. After incubation, 1mL of the pooled sample was aseptically inoculated onto 3 Tryptone Soy Agar (TSA) plates which were used for bacterial analysis (aerobic and anaerobic) and 1 Sabouraud Dextrose Agar (SDA) plate which was used for fungal analysis. The plates were incubated as follows:
1. Bacterial (Aerobic): One TSA plate incubated for 3 days at 30°C to 35°C.
2. Bacterial (Anaerobic): One TSA plate incubated for 3 days at 30°C to 35°C under anaerobic conditions inside an anaerobic gas jar containing an anaerobic gas generator pack and indicator (BD GasPak™ EZ EZ Anaerobe Pouch System).
3. Bacterial (Microaerophilic): One TSA plate incubated for 3 days at 30°C to 35°C under micro- aerophilic conditions inside an anaerobic gas jar cont- aining a microaerophilic gas generator (BD GasPak™ EZ CampyPouch System).
4. Fungus analysis: One SDA plate incubated for 5 days at 20°C to 25°C.
After incubation was completed, the plates were checked for the presence of microbial growth, and the results were reported as CFU/10ml of pooled eggs.
Consignment Number | Number of Pooled Eggs | Consignment Number | Number of Pooled Eggs |
---|---|---|---|
1 | 14 | 10 | 48 |
2 | 17 | 11 | 15 |
3 | 6 | 12 | 15 |
4 | 20 | 13 | 32 |
5 | 17 | 14 | 42 |
6 | 26 | 15 | 16 |
7 | 25 | 16 | 12 |
8 | 54 | 17 | 6 |
9 | 21 |
2.1.3. Sterility Test Methodology
A total of 19 eggs, produced by hens in Ovagen’s Germ Egg Production Facility, were tested according to the sterility test based on the methodology described in chapter 2.6.1 of the European Pharmacopeia [25]. The tests were performed in a Laminar Flow Cabinet. For this test, each egg was tested individually. Nineteen eggs were tested using this methodology as part of our quality control programme. Samples were collected from each avian isolator on a regular basis and tested. On one occasion, one of the eggs broke before testing and for that reason, it could not be tested. At the time of writing, 20 eggs had been collected and 19 were tested as one egg was broken.
The eggshells were disinfected with 70% sterile isopropyl alcohol (IPA) and allowed to dry. The egg was then broken using a sterile spatula, and the content (white and yolk) was aseptically transferred to a sterile sample bag. A total of 50mL of Fluid A (Millipore®: this was used for sample dilution and is the pharmacopoeial solution described in the European Pharmacopoeia chapter 2.6.1) was added to the bag, and it was mixed in a stomacher® for 3 minutes. About 1mL of the egg content was aseptically added to 100mL of TSB (Millipore®: for fungal and aerobic bacterial analysis, according to the European Pharmacopoeia chapter 2.6.1) and Fluid Thioglycollate Medium (FTM) (Millipore®: for aerobic and anaerobic bacterial analysis, according to the European Pharma- copoeia chapter 2.6.1).
TSB was incubated at 20°C to 25°C for 14 days, and FTM was incubated at 30°C to 35°C for 14 days. As the egg renders turbidity to the media, it was not possible to distinguish microbial growth. After 14 days of incubation, a portion of 1mL of each media was transferred to a fresh bottle of the same media and incubated together with the original bottle for 4 additional days under the same conditions described above.
After incubation was completed, the bottles were checked for the presence of microbial growth.
2.1.4. Rapid Microbial Method (bioluminescence)
The chosen method was a qualitative Bioluminescence method where Adenosine Triphosphate (ATP) is detected through an assay using luciferin/luciferase enzymes, which emits light in the proportion of the ATP present. The sample was cultivated in a liquid medium, and the emitted light was measured with a bioluminometer and expressed in relative light units (RLU).
The Bioluminometer used was Promicol Novilite®, and the manufacturer supplied all the reagents and culture media. The culture media used for this test were Tryptone Soy Broth (TSB), Sabouraud Dextrose Broth (SDB), and Anaerobic Tryptone Soy Broth (TSBana) (Promicol®). The tests were performed in a Laminar Flow Cabinet.
A total of 30 eggs, produced by hens in Ovagen’s Germ Egg Production Facility, were tested using the Promicol Novilite® assay. For this test, each egg was tested individually.
The eggshells were disinfected with 70% sterile isopropyl alcohol (IPA) and allowed to dry. Each egg was then broken using a sterile spatula, and the content (white and yolk) was aseptically transferred to a sterile sample bag. A total of 50mL of Fluid A (Millipore®: this was used for sample dilution and is the pharmacopoeial solution described in the European Pharmacopoeia chapter 2.6.1) was added to the bag, and it was placed in a stomacher® for 3 minutes. About 1mL of the egg content mix was added to TSB (Promicol®: for aerobic bacterial analysis), SDB (Promicol®: for fungal analysis), and TSBana (Promicol®: for anaerobic bacterial analysis – this is a Promicol proprietary media). This procedure was repeated for each of the 30 eggs tested.
TSB and TSBana were incubated at 30°C to 35°C for 72 hours. SDB was incubated at 20°C to 25°C for 72 hours. After the incubation period, 100µL of each vessel was added to a microplate in triplicates, and the plate was read on Promicol Novilite®.
2.2. Replication of Human Influenza Virus A/H1N1/Victoria/pdm/2019
The test was conducted by comparing the virus replication of human influenza virus A/H1N1/Victoria/ pdm/2019 in White Leghorn Germ-Free eggs with SPF eggs from 2 different suppliers. The breeds of hens used by SPF supplier 1 and 2 are White Leghorns with different genetic strains. One of these suppliers has a genetic line which is not resistant to any of the Avian Leukosis Virus subgroups, which is important for Leukosis studies and both suppliers meet the requirements of the European Pharmacopoeia Section 5.2.2 “Chicken Flocks Free from Specified Pathogens for the Production and Quality Control of Vaccines”.
Two batches of 15, 10-day-old embryonated eggs from each source were inoculated with ≈100 PFU influenza virus A/H1N1/Victoria/pdm/2019 per embryo and incubated at 37°C under 45% humidity for a total of 72 hours, with a cycle of turning every 30 minutes (Fig. 1).
To serve as controls, 3 eggs from each source were inoculated with phosphate-buffered saline (PBS). Embryo survival was determined by candling every 24 hours.
The allantoic fluid was harvested after 24h, 48, and 72h of infection and quantified by volumetric measurements. Hemagglutination Activity (HA) of the allantoic fluid in each egg was determined using Chicken Red Blood Cells (ChRBC).
The allantoic fluids were clarified by centrifugation at 3,000 rpm for 30 minutes. Positive samples of each group were pooled. The allantoic fluid was then aliquoted and stored at -80°C. Virus titers were determined by plaque assays at 370C for 72 hours.
Further, this test was repeated with an additional batch of 15 Germ-Free eggs.
2.3. Interferon Production Assessment
To investigate if any differences in virus replication kinetics, as well as titers, would be due to lack or reduced innate antiviral responses (IFN-α and IFN-β), an estimate of the IFN-α and IFN-β gene expressions during virus replication using Chick Embryo Fibroblast (CEF) cell culture generated from 10-day-old Germ-Free eggs and from SPF eggs from both suppliers was assessed.
The CEF cells were obtained according to Hernandez and Brown (2010) [26]. A set of 2 chicken embryos from each source was culled at day 10 of embryonation, and the pooled fibroblast cells were seeded into 6-well cell culture plates containing CEF growth media (Medium E199, 10% newborn calf serum, 1% penicillin-streptomycin, 10% Tryptose Phosphate Broth, 0.25% Trypsin-EDTA). The cells were incubated at 37°C under 5% CO2 for 24 hours.
CEF cells were washed with PBS and infected with human influenza virus A/H1N1/Victoria/pdm/2019 at 0.01 multiplication of infection (MOI) for 1 hour and excess virus was removed by aspiration. The cells were washed with PBS and fresh CEF growth media was added. Control wells were inoculated with media only.
After 24h of infection, the supernatant was harvested and titrated for viral HA activity and virus infectivity while cellular RNA extracts were assayed for IFN-α and -β gene expression by RT-qPCR using gene-specific primers and probes (Table 2) that target IFN-α and -β genes in chicken, as well as, a housekeeping gene, RPLO-1, with which the relative gene expressions of IFN-β, and -α were determined using conventional 2−ΔΔCT [27].
An additional experiment was conducted to support these tests with a focus on the replication competence of the Influenza H1N1 Virus in Germ-Free embryonated eggs of the hen and the assessment of interferon responses against Influenza H1N1 in Chicken Embryo Fibroblast (CEF) cells derived from Germ-Free embryonated eggs.
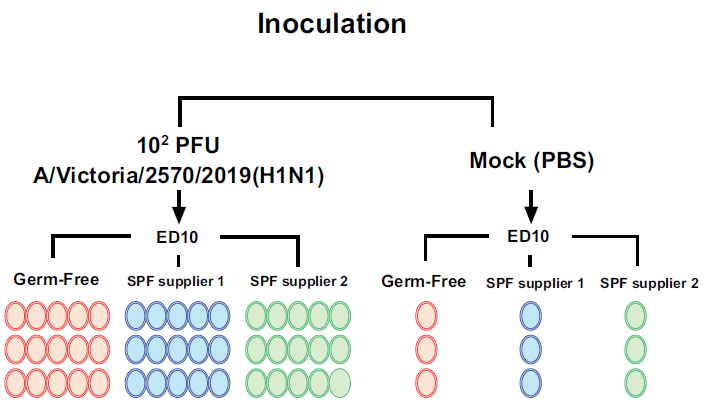
Influenza virus A/H1N1/Victoria/pdm/2019 Virus inoculation scheme to evaluate virus replication in Germ-Free and SPF eggs.
Target Gene | Primer Name | Primer Sequence |
---|---|---|
IFN-α | IFNα FP IFNα RP IFNα probe |
GACAGCCAACGCCAAAGC GTCGCTGCTGTCCAAGCATT FAM-CTCAACCGGATCCACCGCTACACC-TAMRA |
IFN-β | IFNβ FP IFNβ RP IFNβ probe |
CCTCCAACACCTCTTCAACATG TGGCGTGTGCGGTCAAT FAM-TTAGCAGCCCACACACTCCAAAACACTG-TAMRA |
28S rRNA | 28S FP 28S RP 28S probe |
GGCGAAGCCAGAGGAAACT GACGACCGATTTGCACGTC FAM-AGGACCGCTACGGACCTCCACCA-TAMRA |
RPLPO-1 | RPLPO-1 FP RPLPO-1 RP RPLPO-1S probe |
TTGGGCATCACCACAAAGATT CCCACTTGTCTCCGGTCTTAA FAM-CATCACTCAGAATTTCAATGGTCCCTCGGG-TAMRA |
2.4. Influenza Virus Plaque Assay
Plaque assays were carried out to titrate human influenza A virus (IAV) A/H1N1/Victoria/pdm/2019 (pdm019) propagated in allantoic fluid of embryonated hens’ eggs. Briefly, confluent Madin-Darby Canine Kidney (MDCK) cells (CCL-34™, American Type Culture Collection, Manassas, VA, USA) were seeded in 12-well plates and cultured in Dulbecco’s Modified Eagle’s medium (DMEM) (Merck Life Science), supplemented with 100 U/mL penicillin, 100μg/mL streptomycin (Gibco, Life Techno- logies), and 10% Foetal Calf Serum (FCS) (Merck Life Science), at 37°C with 5% CO2. The cells were infected with 10-fold serially diluted pdm 09 at 250 µl per well and incubated for one hour at 37°C. The virus inoculum was removed, and 1mL of overlay medium (see below) containing 2 µg/mL TPCK-treated trypsin and 1% melted agarose (Oxoid Life Technologies) was added at 37°C and allowed to set. The plates were inverted and incubated for 3 days at 37°C or until visible plaques were formed. The agar discs were gently removed from the wells, and each well was stained with a 0.1% crystal violet solution (Merck Life Science) (40 mL 1% crystal violet solution, 80 mL methanol, 280 mL water) for at least 30 minutes. The stained monolayer was then washed gently with water and air-dried. The virus was titrated to plaque-forming units (pfu)/mL by counting the dilution of distinguishable plaques at the lowest dilution (with at least 10 in the well) and multiplying by virus inoculum dilution factor (250 µl x4- 1mL).
The overlay media contains 100mL of 10x MEM (Modified Eagle’s Medium) (Merck Life Science), 28 mL of 7.5% filtered BSA (Merck Life Science), 10 mL of 200 mM L-Glut in saline (Merck Life Science), 20 mL of 7.5 NaHCO2 (Gibco, Life Technologies), 10 mL 1M Hepes (Gibco, Life Technologies), 5 mL of 1% dextran hydrochloride (Merck Life Science), 10 mL of 10x Pen/Strep (Gibco, Life Technologies), 517 mL of water.
Pooled Eggs | Bacteriology Analysis* | Fungal Analysis |
---|---|---|
14 | No growth | No growth |
17 | No growth | No growth |
6 | No growth | No growth |
20 | No growth | No growth |
17 | No growth | No growth |
26 | No growth | No growth |
25 | No growth | No growth |
54 | No growth | No growth |
21 | No growth | No growth |
48 | No growth | No growth |
15 | No growth | No growth |
15 | No growth | No growth |
32 | No growth | No growth |
42 | No growth | No growth |
16 | No growth | No growth |
12 | No growth | No growth |
6 | No growth | No growth |
3. RESULTS
3.1. Microbiological Analysis by Independent Laboratory
A total of 386 eggs were sent to an independent specialized contract laboratory - Complete Laboratory Solution (CLS), Galway, Ireland, to be tested for the presence of bacteria and fungi.
All 386 eggs presented with no growth in all tested media. The results are presented in Table 3.
3.2. Sterility Test Methodology
A total of 19 eggs were tested for sterility using the methodology based on chapter 2.6.1 of the European Pharmacopeia. From the 19 tested eggs, 1 egg presented growth in TSB and FTM media after 72h of incubation.
The vessel containing bacterial growth was subcultured to TSA, and the micro organism was gram-stained. In addition, gram-negative rods were detected.
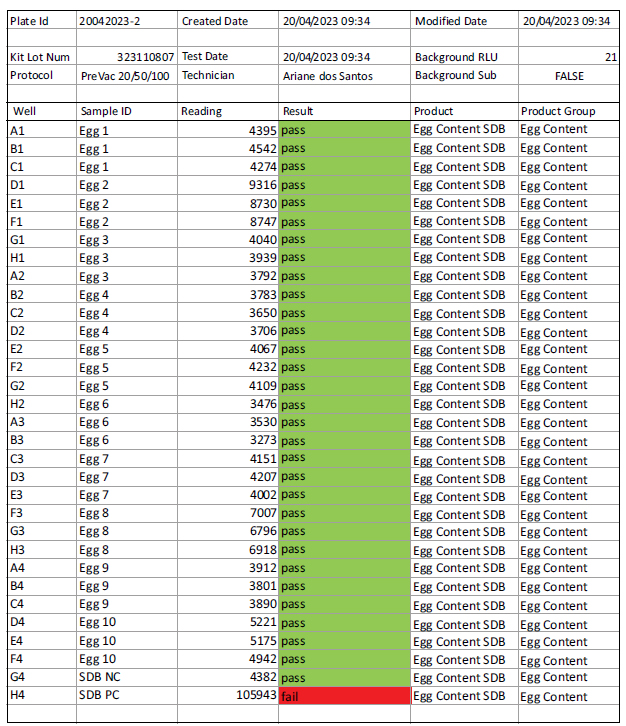
Sample report of Promicol Novilite® assay. Pass – no contamination detected / Fail – contamination detected.
3.3. Rapid Microbial Method (bioluminescence)
A total of 30 individual eggs were tested using the Promicol Novilite® assay. In this methodology, the samples were incubated for 72h, and then a bioluminescence assay was performed.
All 30 individual egg samples passed the Promicol assay, which means no microbial ATP was found. Fig. (2) presents a sample report of the Promicol Novilite® assay.
3.4. Replication of Human Influenza Virus A/H1N1/Victoria/pdm/2019
The allantoic yield of embryos was similar between groups at the same time point, with each group displaying a non-significant increase in yield every 24 hours (p>0.05) (Fig. 3).
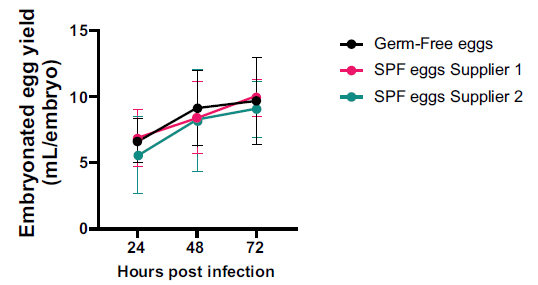
Comparative allantoic volume recovered from germ-free and SPF eggs. The data are presented as mean±SD (n=10) and analysed by two-way ANOVA with Šidák and Tukey’s multiple comparison test.
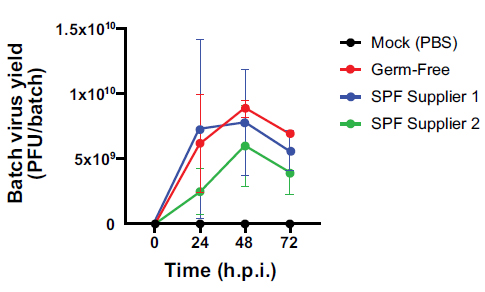
Comparative viral yield from germ-free and SPF eggs. Viral yield at 24h, 48h, and 72h for the GF eggs and the SPF eggs from two different suppliers. The data are presented as mean±SD (n=2) and analysed by two-way ANOVA with Šidák and Tukey’s multiple comparison test.
The peak virion yield time points were the same for all groups of eggs tested at 48h after infection. The pooled allantoic fluid collected from 5 Germ-Free eggs after 48 hours of infection presented more virion particles than batches of 5 SPF eggs, with the number of particles detected from Germ-Free eggs being ~1.1-fold and ~1.5-fold higher than SPF Supplier 1 and SPF Supplier 2, respectively (p>0.05).
After 72h of infection, the batch yield of 5 Germ-Free eggs was ~1.2-fold and ~1.8-fold higher than SPF supplier 1 and SPF supplier 2 virus yields, respectively (p>0.05) (Fig. 4).
There was no significant difference in virion particles in the allantoic fluid between study 1 and the additional experiment using Germ-Free eggs (Fig. 5). In the original experiment, optimal replication at 48 hrs was 8.87x109 pfu/batch. In the additional experiment, optimal replication at 48 hrs was 1.11x109 pfu/batch. Less than 1 log10 differences between batches are considered non-significant due to technical repeat differences, such as variation in the inoculum titres during the preparation of inoculum.
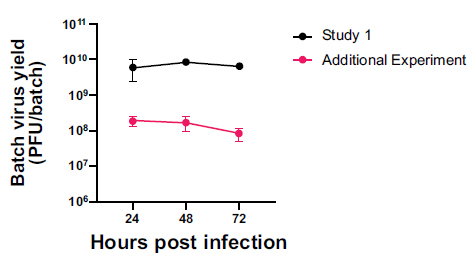
Comparative viral yield from Germ-Free eggs in the first study and the repetition. The data are presented as mean±SD (n=2) and analysed by two-way ANOVA with Šidák multiple comparison test.
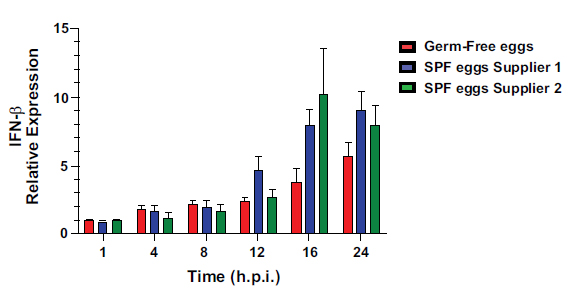
Interferon Beta (IFN-β) comparison. Levels of IFN-β expression at 1h, 4h, 8h, 12h, 16h, and 24h for the CEF cell culture from GF eggs and the SPF eggs from two different suppliers.
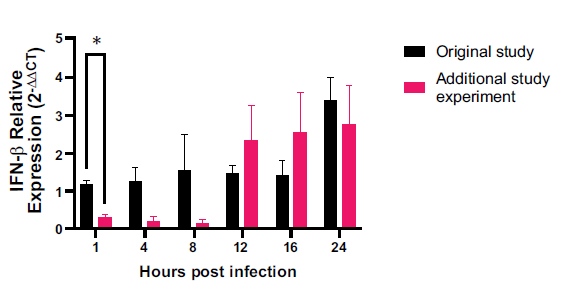
Interferon Beta (IFN-β) comparison. Levels of IFN-β expression at 1h, 4h, 8h, 12h, 16h and 24h for the CEF cell culture from GF eggs in the first experiment and the additional study. The data are presented as mean±SD (n=3) and analysed by two-way ANOVA with Šidák multiple comparison test.
3.5. Interferon Production Assessment
Using CEF (Chick Embryo Fibroblasts) derived from Germ-Free hatching eggs, replication of human influenza virus A H1N1/Victoria was 2-fold higher than CEF derived from SPF supplier 1 or SPF supplier 2 eggs (p<0.001).
IFN-β antiviral response of Germ-Free-derived CEF cells was 2-fold lower than SPF supplier 1-derived and ~3-fold lower than SPF supplier 2-derived CEF cells (p<0.05) (Fig. 6).
A significant difference was observed between experiment 1 and the additional study experiment on IFN-β only at the timepoint of 1hpi (p<0.05), in which the levels of IFN-β were significantly lower in the additional study than it was in the first one (Fig. 7). There was no significant difference in IFN-β expression in response to influenza H1N1 Victoria infection between study 1 and the additional experiment at the remaining time points (4-24hpi).
4. DISCUSSION
In this paper, we present the results of the analyses of the contents of 445 Germ-Free eggs. Three different methods were used by two different laboratories to analyze the contents of the eggs. From those, only one egg, representing 0.2% of the total number of eggs tested in this study, showed evidence of bacterial growth.
This contamination was most likely a false positive carried from the exterior of the egg/the environment into the internal contents of the egg during processing. One limitation of this study is that this contamination was not further investigated in terms of the identification of the gram-negative rods detected.
The power calculation showed that 160 eggs would be required to disprove the null hypothesis that the proportion is greater than 0.0001% (one in one million). This study analyzed the contents of 445 eggs, of which 444 were free of microbial growth. This probability of less than one out of one million was used in this study because that is the parameter established by the World Health Organization for the pharmaceutical industry to define a container as sterile [29].
In all instances, the combined results of the analyses, i.e., total viable count, sterility test, and bioluminescence confirmed that the contents of the eggs were sterile. Furthermore, an independent analysis of the contents of the Germ-Free eggs was carried out by a global vaccine manufacturer confirming that the eggs were sterile on arrival and also after 11 days of incubation (data not presented). These data also show the effectiveness of the egg packaging system in maintaining the sterility of the eggs during transport.
Luminescent reactions, where structures of both luciferin and luciferases have been discovered, are now utilized in vitro and in vivo in food testing [30], environ- mental monitoring [31], diagnostics [32], drug screenings [33-35], and various kinds of biomedical research. The use of bioluminescence to detect adenosine triphosphate (ATP) is a very robust technique to determine if the contents of eggs are sterile as it detects ATP from micro-organisms in the egg content.
Another of the potential limitations of this study relates to the microbiological analysis of eggs for the presence of bacteria and fungi using the microbial enumeration tests approach wherein the contents of variable numbers of eggs were pooled, which is a methodology designed for non-sterile products. While this may be considered a limitation from a methodological perspective, the analysis of eggs using bioluminescence, which can be considered the most robust measurement of sterility, failed to find any growth in the contents of the Germ-Free eggs analyzed. These three different methods for microbiological analysis of egg contents and the independent vaccine manufacturer's confirmation of the same clearly demonstrate the sterile nature of the contents of Germ-Free eggs.
Interferons, a subclass of cytokines, are produced in the body during illnesses, such as influenza, in order to help fight the infection. They are responsible for many of the symptoms of influenza infections [28], including fever, muscle aches, fatigue, and headaches. In that context, interferons can be viewed as an important part of the defense mechanism of the body against viral disease(s). One of the key objectives of this study was to determine if an increase in viral yield of the human influenza virus could be attributable to reduced levels of interferon.
The results of this initial study showed that when Human Influenza Virus A/H1N1/Victoria/pdm/2019 was inoculated into Germ-Free eggs, a non-significant two-fold increase in viral yield was achieved when compared with eggs from SPF flocks from two different suppliers. The increase in viral yield was associated with a significant, almost three-fold reduction in interferon Beta levels, which supports our hypothesis.
To the best of our knowledge, this is the first time that germ-free eggs have been used for this purpose, and these data show an important potential application for germ-free eggs in vaccine manufacturing. We are currently in the process of performing such trials with a number of global vaccine manufacturers.
The implications of an increased yield of virus/vaccine from eggs will also have significant societal and economic benefits as more vaccines can be produced from eggs at a reduced cost, thereby providing the opportunity for more widescale production of human and animal vaccines from eggs [36] with a possible positive effect on the immunogenicity of influenza viruses [37, 38]. The sample size was small in this study, which can be viewed as a potential limitation of the study. Due to this, an additional study was performed with Germ-Free eggs. The results of the additional study endorsed the results of the first study with similar viral yield and IFN-β expression. Our results corroborated the rodent studies conducted by Ichinohe et al., where reduced interferon levels were associated with an increase in viral yield [22].
Three additional studies are planned to further assess the comparative viral yield and INF-β expression of Newcastle Disease virus, Yellow Fever virus, and Newcastle Disease Virus (NDV)-vectored COVID-19 virus when inoculated into Germ-Free eggs and SPF eggs.
CONCLUSION
The results of this study show that the contents of 445/446 Germ-Free eggs were sterile, supporting the hypothesis that the proportion of microbiologically contaminated eggs is at most 0.0001%, which complies with the World Health Organization standard for pharmaceutical practice to define a container as sterile.
The yield of Human Influenza Virus A/H1N1/ Victoria/pdm/2019 was higher in Germ-Free Eggs when compared to SPF eggs, and this higher yield was associated with a significant reduction in interferon Beta levels. Moreover, further studies are planned to determine the viral yield of Germ-Free eggs after inoculation with Newcastle Disease virus, Yellow Fever virus, and Newcastle Disease Virus (NDV)-vectored COVID-19 virus.
AUTHORS’ CONTRIBUTIONS
A-d.S.: Contributed to the conceptualization, methodology, data acquisition, analysis, interpretation, validation, original draft writing, review, and editing; M.M.: Contributed to the original draft writing, review, and editing; M.A., O.A., T.C., J-R.S., M.I.: Contributed to the methodology, data acquisition, analysis, interpretation, original draft writing, review, and editing; C.C., L.M.: Contributed to the original draft writing, review, and editing.
LIST OF ABBREVIATIONS
CEF | = Chick Embryo Fibroblasts |
CLS | = Complete Laboratory Solutions |
CFU | = Colony-forming unit |
FTM | = Fluid Thioglycollate Medium |
IFN- α | = Interferon Alpha |
IFN-β | = Interferon Beta |
HA | = Haemagglutinin |
IPA | = Isopropyl alcohol |
MOI | = Multiplicity of infection |
NS1 | = Non-structural protein-1 |
SPF | = Specific Pathogen Free |
PBS | = Phosphate-buffered Saline |
PFU | = Plaque Forming Unit |
RNA | = Ribonucleic acid |
SDA | = Sabouraud Dextrose Agar |
SDB | = Sabouraud Dextrose Broth |
TSA | = Trypticase soy agar |
TSB | = Tryptone Soy Broth |
TSBana | = Anaerobic Tryptone Soy Broth |
TVC | = Total Viable Count |
ETHICAL STATEMENT
All work involving embryonated eggs was carried out in strict accordance with European and United Kingdom Home Office legislation. The in ovo work undertaken in this study was reviewed and approved by the Pirbright Institute Animal Welfare and Ethical Review Body to comply with the relevant UK legislation, in accord with the UK Home Project License PP6471846. All experiments involving infection of embryonated hen eggs or cultured cells with influenza virus were undertaken in a licensed biosafety level 2 conditions.
AVAILABILITY OF DATA AND MATERIALS
The authors confirm that the data supporting the findings of this study are available within the article.
FUNDING
This work was financially supported by the European Innovation Council (EIC) 2022 Accelerator Fund 9grant number 190136873).
CONFLICT OF INTEREST
The authors confirm that there is no conflict of interest, financial or otherwise.
ACKNOWLEDGEMENTS
The authors would like to thank the Pirbright Institute’s research team (Munir Iqbal, Jean-Remy Sadeyen, Toby Carter, and Oluwapelumi Adeyemi) for their support with the viral yield and interferon investigations. The work was partly funded by the UKRI-BBSRC grants numbers BBS/E/PI/230001A, BBS/E/PI/ 230001B, BBS/E/PI/230001C, BBS/E/PI/23NB0003.
They would like to thank Promicol for the kind supply of Promicol Novilite® Bioluminometer, all the necessary media and reagents, and their full support during this study.
They would also like to thank the CLS microbiology team for their support with the microbiological analysis of the eggs.