All published articles of this journal are available on ScienceDirect.
Identification of Klebsiella Variicola T29A Genes Involved In Tolerance To Desiccation
Abstract
Introduction:
Several plant-beneficial bacteria have the capability to promote the growth of plants through different mechanisms. The survival of such bacteria could be affected by environmental abiotic factors compromising their capabilities of phytostimulation. One of the limiting abiotic factors is low water availability.
Materials and Methods:
In extreme cases, bacterial cells can suffer desiccation, which triggers harmful effects on cells. Bacteria tolerant to desiccation have developed different strategies to cope with these conditions; however, the genes involved in these processes have not been sufficiently explored. Klebsiella variicola T29A is a beneficial bacterial strain that promotes the growth of corn plants and is highly tolerant to desiccation. In the present work, we investigated genes involved in desiccation tolerance.
Results & Discussion:
As a result, a library of 8974 mutants of this bacterial strain was generated by random mutagenesis with mini-Tn5 transposon, and mutants that lost the capability to tolerate desiccation were selected. We found 14 sensitive mutants; those with the lowest bacterial survival rate contained mini-Tn5 transposon inserted into genes encoding a protein domain related to BetR, putative secretion ATPase and dihydroorotase. The mutant in the betR gene had the lowest survival; therefore, the mutagenized gene was validated using specific amplification and sequencing.
Conclusion:
Trans complementation with the wild-type gene improved the survival of the mutant under desiccation conditions, showing that this gene is a determinant for the survival of K. variicola T29A under desiccation conditions.
1. INTRODUCTION
The diversity of bacteria is greater than that of any other group of organisms [1], and many bacterial species have beneficial properties for plants [2-5]. These beneficial bacteria can promote plant growth through different mechanisms, such as biological nitrogen fixation, phytohormone production and phosphate solubilization [6-10]. They can also produce a beneficial effect by antagonizing pathogenic microorganisms of the plant [3, 11-13], remediating heavy metals [14, 15] and degrading xenobiotic compounds [16, 17]. The survival of beneficial bacteria may be limited by environmental abiotic factors, such as the stress imposed by low water availability [18]. Water is a determining factor for the life of living beings, and the limitation of this resource causes the dehydration of bacterial cells and, in extreme conditions, desiccation [19]. which is defined as the loss of water from cells, until equilibrium with the water present in the air is reached [20]. Under desiccation conditions, the water content inside cells decreases until it reaches <0.1 grams of water per gram of dry weight (<10%) at 50% relative humidity and 20°C [20, 21].
Under desiccation conditions, bacteria suffer several alterations, such as DNA, protein and membrane damage; changes in membrane permeability; Maillard reactions; and generation of reactive oxygen species [21-23]. To tolerate stress caused by desiccation, it has been observed that some bacterial strains enter a dormant state or a viable but nonculturable state [24-27], which allows them to survive once the stress conditions have ended and the bacteria find favorable conditions to divide again.
Some of the strategies used by bacteria to tolerate desiccation are related to the synthesis of highly hydrophilic proteins [28, 29], changes in the cellular envelope [30], synthesis and accumulation of carbohydrates as trehalose [31-34], repair of genetic material and polysaccharide production [35-37] and biofilm formation [30, 38, 39]. Furthermore, overproduction of DNA has been associated with the protection of bacterial cells from desiccation [40].
Some genes are related to bacterial desiccation tolerance. For example, those encoding proteins with regulatory functions (RelA, RpoE2 and Hpr) and DNA repair (UvrC) in Sinorhizobium meliloti [41]. Genes encoding proteins involved in the biosynthesis of the flagellum (fliP, flhB, flgD, flgL), motor control of the flagellum (motB, fliM, fliY), membrane lipid biosynthesis, energy production, potassium incorporation and virulence are involved in desiccation tolerance and osmotic stress in Listeria monocytogenes [42]. The treS gene encodes a protein involved in the biosynthesis of trehalose, an important disaccharide to tolerate desiccation in Bradyrhizobium japonicum [43]. sopD and sseD genes encode virulence factors that have been associated with desiccation tolerance in Salmonella enterica [32].
Transcriptomic studies have revealed that desiccation conditions trigger the expression of several genes, such as those genes which encode proteins related to antimicrobial resistance, extrusion pumps and quorum quenching in Acinetobacter baumannii [25]. Additionally, genes encoding proteins related to trehalose and polysaccharide biosynthesis and genes encoding proteins involved in membrane protection, DNA repair, protein stability and integrity, response to oxidative stress and chaperones were observed in Bradyrhizobium japonicum [35, 44]. The expression of genes encoding proteins related to alginate and flagellum synthesis has been observed in Pseudomonas putida KT2440 under desiccation conditions [45]. An increase in gene expression related to metabolic pathways, transporter regulation, DNA repair and replication, transcription, translation and virulence occurred in Salmonella enterica [32]. In addition, some genes encoding proteins involved in protection under oxidative stress (dps1) and genes encoding sigma factors, i.e., SigF1 and SigF3, were positively regulated in Rhodococcus jostii RHA1 under desiccation conditions [46].
In previous studies, the ability of different rhizospheric bacterial strains to tolerate desiccation has been explored, and five levels of bacterial tolerance were established: high tolerance (BSRad>80), tolerance (60<BSRad≤80), moderate tolerance (40<BSRad≤60), low tolerance (20<BSRad≤40) and very low tolerance (BSRad≤20) [47]. Klebsiella variicola T29A is a bacterial strain isolated from the sugar cane stem [48] that promotes the growth of corn plants [47]. In K. variicola T29A, direct mechanisms of plant growth-promotion have been reported, such as indole-3-acetic-acid production, solubilization of phosphates, production of gibberellins; and indirect mechanisms such as siderophore production [49]. This bacterial strain is tolerant to desiccation and can phytostimulate plants even if the inoculated seeds are subjected to desiccation for 18 days [47]. In the present study, we constructed a transposon mutant library by random mutagenesis to screen for tolerance to desiccation. Mutants sensitive to desiccation were selected to identify genes of K. variicola T29A encoding proteins involved in desiccation tolerance.
2. MATERIALS AND METHODS
2.1. Bacterial Strains and Culture Conditions
The strains used in the present work and the culture media used for bacterial growth are shown in Table 1. Bacterial growth was carried out at 30°C for all the tests in this work. The antibiotics added to the media varied according to the type of test; this is clarified in the corresponding section.
2.2. Generation of K. variicola T29A Mutant Library by Random Transposition Of The Mini-Tn5
Triparental conjugation was performed using E. coli CC118 λpir as a donor strain of the pUT mini-Tn5Km plasmid, K. variicola T29A as the recipient and E. coli RK600 as the helper. For conjugation, each strain was grown in 10 mL of LB medium and incubated overnight at 30°C with constant agitation (200 rpm). Then, the cells were washed two times by centrifugation, the supernatant was eliminated and the cells were resuspended in the same volume of sterile distilled water. Subsequently, a mixture was made using 500 μL of each strain, and a drop of 20 µL was placed on the edge of a plate of MESMA gel medium (Table 1), which was incubated for 24 h at 30°C. Bacteria were collected using 3 mL of a 10 mM MgSO4 solution. The same procedure was followed for individual cultures of each of the 3 control strains. From the obtained bacterial suspensions, 1:10 serial dilutions were made, and 100 μL of each dilution was plated on 4 different culture media, including selective medium MM9/sodium citrate containing 80 μg/mL of kanamycin (Km80) (to select K. variicola mutants), LB-Km80 (to select E. coli cc118λpir), LB containing 30 μg/mL chloramphenicol (Cm30) (to select E. coli RK600) and MM9-sodium citrate (to select wild-type K. variicola T29A), which were incubated for 24 h. Iindividual colonies of K. variicola T29A that had acquired mini-Tn5 transposon and grew in the MM9-sodium citrate-Km80 selection medium [51] were transferred to plates of the same medium to verify their resistance to the antibiotic and the clones that grew were stored individually at -80°C, in 25% glycerol.
Bacterial Strain | Relevant Characteristics | Culture Medium Used in this Work | Purpose |
---|---|---|---|
Klebsiella variicola T29A | Isolated from sugar cane [48], highly tolerant to desiccation and promoter of plant growth [47] | MacConkey (casein peptone 1.5 g/L, gelatin peptone 17 g/L, meat peptone 1.5 g/L, lactose 10 g/L, bile salts 1.5 g/L, Sodium Chloride 5 g/L, neutral red 0.03 g/L, crystal violet 0.001 g/L, agar 13.5 g/L) | Selection medium |
MESMA (glucose 2.7 g/L, mannitol 1.8 g/L, yeast extract 2.7 g/L, MES 4.4 g/L, KH2PO4 0.65 g/L, K2HPO4 4.8 g/L) [50] | Growth medium for desiccation assays | ||
MM9-Citrate [51] | Medium used in random mutagenesis assays | ||
Escherichia coli CC118 λpir | Strain (λpir +), donor of the pUT mini-Tn5Km plasmid. The plasmid only self-replicates in the presence of the λpir protein and is suicidal in other genetic backgrounds. | LB (casein peptone 10 g/L, yeast extract 5 g/L and NaCl 10 g/L) -kanamycin containing 80 μg/mL of antibiotic (LB-Km80) [52] | Medium used in random mutagenesis assays |
Escherichia coli RK600 | Helper strain, contains plasmid RK600, tra + | LB-chloramphenicol (LB-Cm30) | The medium used in random mutagenesis assays |
Klebsiella variicola T29A::mini-Tn5Km (from mutant 1 to 8974) | Mutants that acquired the mini-Tn5Km transposon in some region of its genome | MM9-Citrato-Km80 | The medium used for the selection of mutants that acquire the mini-Tn5Km transposon |
For each conjugation experiment, the number of CFU/mL in the mixture and in the plates of selective medium was quantified by the drop plate method [53], and the transposition rate was calculated using the relation [(CFU/mL) in the selection medium/(CFU/mL) in the mixture]. Some mutants from different conjugation events were selected, and their plasmid profile was determined to ensure that they did not contain the plasmid pUT mini-Tn5Km; consequently, the transposon mini-Tn5Km was integrated into the bacterial chromosome. The extraction of plasmids was carried out on overnight cultures of the strains using a “Plasmid Purification” kit (QIAGEN), and electrophoretic shift was performed on a 1% agarose gel.
2.3. Desiccation Tests for the Identification of Sensitive Mutants
The mutant library was used to identify T29A mutants sensitive to desiccation by performing a massive desiccation experiment. The wild-type strain and the 8974 mini-Tn5 mutants were inoculated in the PY-Calcium medium and incubated under agitation at 30°C until they reached a stationary phase. Cells were washed twice by centrifugation and resuspended in the same volume of sterile distilled water, and 100 μL of each mutant sample was added to a 96-well plate. The plates were placed in a drying chamber for 18 days at 30°C and 50% relative humidity. The samples were desiccated completely on the third Day After the Beginning of Desiccation (DABD). The number of CFU/mL was determined by the Massive Stamping Drop Plate (MSDP) method [7, 54, 55], both before desiccation and at 18 DABD. This allowed us to calculate the Bacterial Survival Rate (BSR), which is defined as the ratio of the log of the number of bacterial cells present in the suspension at any given time Post Desiccation (PD) in relation to the log number of viable cells before desiccation (BD), all multiplied by 100; BSR= [(logPD + 1)/logBD]×100 [7, 56, 57]. Mutants in which the BSR was diminished with respect to the wild strain were selected and considered “presumptively sensitive”. These mutants were used to carry out experiments to validate the sensitivity to the desiccation phenotype.
2.4. Validation of Desiccation Sensitivity of K. variicola Mutants
Overnight cultures of wild-type and selected mutants were inoculated in 50 mL of MESMA medium with an initial optical density of 0.05 at 620 nm. Bacterial cells grown in the stationary phase were centrifuged (5000 rpm for 10 minutes), washed and resuspended in sterile distilled water. For each bacterial suspension, 35 aliquots of 500 μL were dispensed in Eppendorf tubes with a capacity of 1.5 mL, which were covered with cotton plugs to allow the evaporation of water. Five samples were used to quantify the number of bacteria existing before desiccation using the MSDP method in the McConkey-Km80 medium (Bioxon). The remaining aliquots were placed in a drying chamber (30°C and 50% relative humidity) for 18 days. Under these conditions, samples were completely desiccated at 5 DABD (not shown). For every 3 DABD, 5 samples were taken, rehydrated with distilled water for 20 minutes, and the number of CFU/mL was quantified using the MSDP method [http://dx.doi.org/10.17504/protocols. io.j4icque]. The BSR was calculated for each of the experimental samples. It should be noted that the weight of the tubes was verified before and after the desiccation process as a parameter to determine the water loss of the samples and to guarantee a state of desiccation.
2.5. Determination of the Interrupted Genes in Mutants Sensitive to Desiccation by Arbitrary PCR
The amplification of the kanamycin resistance gene found in the mini-Tn5 transposon was carried out using genomic DNA from validated desiccation-sensitive mutants to verify whether this transposon was inserted in these mutants. The following oligonucleotides were used: mini-Tn5KmFw and mini-Tn5KmRv (Table 2). DNA from the wild-type strain was used as a negative control. The amplification product was verified by electrophoresis on 1% agarose gel and 1% TAE (tris-acetate-EDTA).
Interrupted genes from desiccation-rehydration sensitive mutants were determined by the arbitrary PCR method [42], which allowed us to determine the sequence of nucleotides that flanked the insertion site of the mini-Tn5 transposon. In the first round of PCR, amplifications were performed in a final volume of 50 μL with 100 ng template DNA using the GoTaq® Green Master Mix kit (Promega) by following the indications of the manufacturer. PCR reactions were performed with the genomic DNA of K. variicola T29A and the desiccation-rehydration sensitive mutants, using the following oligonucleotides: specific primer designed to anneal to 3’-end of transposon miniTn5, and semi-degenerate primers ARB1A and CEKG2B (Table 2). In the second round of PCR, the amplification product of the first round was used as a DNA template and amplification was performed using the following oligonucleotides: a specific nested primer TNINT and the primer ARB2A, complementary to the sequence shared by the two random primers (Table 2).
Thirty microliters of the amplification products from the second round of arbitrary PCR were separated on a 2% agarose gel. After this electrophoresis, the most abundant band with an average size of 200 to 600 bp was selected and used to purify the DNA using a “Zymoclean TM Gel DNA Recovery Kit” (ZYMO RESEARCH). Samples were sequenced by Instituto de Biotecnología, Universidad Nacional Autónoma de México. The obtained sequences were aligned with the genome sequence of K. variicola T29A (GenBank, accession number: CXPA00000000.1) using BLASTX software (National Center for Biotechnology Information https://blast.ncbi.nlm.nih.gov).
The mutant with the BSR=0 (KvDSM 6) was selected to validate the insertion site of the mini-Tn5 transposon, which was determined by two PCR rounds with specific primers. For this, 2 amplifications were carried out by PCR using DNA from the wild-type and mutant strains as templates and 2 pairs of oligonucleotides. In the first reaction, a specific oligonucleotide of the transposon was used in the complementary chain (Tn5-Rv) and one of the ends of the gene (Mut 6 stop-Fw). In the second PCR, a transposon-specific oligonucleotide was used in the leader chain (Tn5-Fw) and in the designed oligonucleotide 223 base pairs upstream of the start of the gene (Mut 6 start-Rv) (Fig. 1); (Table 2). The term Mut 6 refers to specific oligonucleotides that amplify DNA regions of 695 bp and 490 bp of the mutant KvDSM 6 (Fig. 1). The PCR products of the two reactions were subjected to electrophoresis on agarose gel (1%) to verify the size of the amplification products. These products were purified by a “ZymocleanTM Gel DNA Recovery Kit” (ZYMO RESEARCH) and sent to be sequenced. The sequences (Supplementary Table 1) were compared with the NCBI databases using BLASTX software (National Center for Biotechnology Information https://blast.ncbi.nlm.nih.gov).
Primers | Sequence (5' → 3') | Annealling Temperature (°C) |
---|---|---|
mini-Tn5KmFw | TATGCCTCTTCCGACCATCA | 61 |
mini-Tn5KmRv | CCGACTCGTCCAACATCAAT | |
ARB1A | CCACGCGTCGACTAGTACNNNNNNGATAT | 40 |
TNEXT2 | CTTTATTGATTCCATTTTTACACT | |
CEKG2B | GGCCACGCGTCGACTAGTACNNNNNNNNNNACGCC | |
ARB2A | CCACGCGTCGACTAGTAC | 60 |
TNINT | GACCTGCAGGCATGCAAGCTTCGGC | |
Tn5-Rv | GGTACCGAGCTCGAATTCGG | 60 |
Mut 6 stop-Fw | CAGTTTTCATGTTCGGTTTC | |
Tn5-Fw | GACCTGCAGGCATGCAAGCTTCGGC | 60 |
Mut 6 start-Rv | AGTCATGCTCCTTCAGGAAC | |
Mut 6 start-Rv | ATGGTACCAGTCATGCTCCTTCAGGAAC | 64 |
Mut 6 stop-Fw2 | CCGAGCTCATTCCAATTATAGAATCACC | |
pJET1.2 forward sequencing primer | CGACTCACTATAGGGAGAGCGGC | 60 |
pJET1.2 reverse sequencing primer | AAGAACATCGATTTTCCATGGCAG |
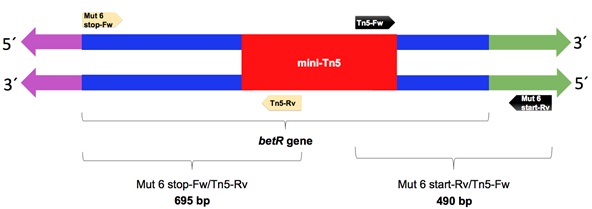
2.6. betR Gene Complementation Assays
A pair of oligonucleotides (Mut 6 start-Rv and Mut 6 stop-Fw2; Table 2) was designed to amplify the betR gene, including 223 bp upstream of the gene and 228 bp downstream of the ORF terminus, in accordance with the sequence of the betR gene of K. variicola T29A (emb|CTQ00968.1=). An expected product of 1297 base pairs was amplified from the genomic DNA of the wild-type strain. The amplified DNA fragment that includes the BetR gene was amplified with the enzyme Taq Polymerase and the Blunt-End cloning protocol from the CloneJET PCR Cloning kit (Thermo Scientific) was used to leave the ends of the amplified DNA in blunt (because Taq polymerases leaves a terminal adenine not paired at the ends 3'). Subsequently blunt-ended product was cloned (using T4 DNA ligase) into the pJET1.2/blunt cloning vector (Supplementary Fig. 1), which was provided by the Clone JET PCR Cloning kit in a linearized form with blunt ends. The ligation product was transformed into E. coli DH5α by heat shock, and the transformants were selected in an LB medium supplemented with ampicillin at a concentration of 200 μg/mL (Amp200). The pJET1.2-BetR construct plasmid was purified from E. coli DH5α, transferred by this same technique to the mutant K. variicola T29A::mini-Tn5Km, inserted into the betR gene (KvDSM 6 mutant), and the transformants were selected in McConkey-medium plates with Amp200. The construct was confirmed by sequencing cloned fragments using a pJET1.2 forward sequencing primer and a pJET1.2 reverse sequencing primer (Table 2).
Subsequently, the recovery of the desiccation-rehydration resistance phenotype was evaluated. For this purpose, the complemented mutant was subjected to desiccation for 18 days under the conditions previously described, and after rehydration, the BSR was determined. In this test, the wild-type strain and mutant KvDSM 6 were included as controls.
3. RESULTS
3.1. Mutagenesis by Random Transposition of the Mini-Tn5Km
A mini-Tn5Km transposon mutant library was constructed in K. variicola T29A as described in the Materials and Methods. The transposition rate was 1.5 x 10-4, which means that transposition occurred in 3 out of every 200 bacteria. The final library contained 8974 mutants.
To verify the excision of the mini-Tn5-containing plasmid (pUT mini-Tn5Km), some mutants were randomly selected for testing; and the plasmid was not identified in any of them, suggesting that the integration of the mini-Tn5 transposon into the chromosome of the bacterium occurred very efficiently. In fact, the pUT mini-Tn5Km plasmid was observed only in the strain E. coli CC118 λpir that was used as a donor for the conjugation experiments and not in the mutants (Supplementary Fig. 2). It is important to note that K. variicola T29A possesses a natural plasmid with a size greater than 10 kb that is observed in mutant strains (Supplementary Fig. 1).
3.2. Desiccation Tolerance in Mutants of K. variicola T29A: Mini-Tn5Km
After evaluating the survival of 8974 mutants under desiccation conditions using a rapid methodology, it was observed that 25 mutants presented a lower BSR with respect to the wild-type strain at 18 DABD, and they were selected for the next round of experiments (Fig. 2). These mutants were subjected to validation experiments to determine their desiccation sensitivity phenotype using a methodology with higher sensitivity [7]. Several replications were performed and the bacterial survival ratio was calculated during the time in which the different mutants were subjected to desiccation (Fig. 3). It was observed that 14 mutants showed a significant decrease in the BSR with respect to the wild strain after being subjected to desiccation for 18 days. These mutants were characterized by their different levels of tolerance; the most affected mutants, KvDSM 6 and KvDSM 12-93, were considered less tolerant to desiccation (BSRad≤20). Low-tolerance was observed in the KvDSM 1-29, KvDSM 9-20, KvDSM 12-43, KvDSM 17-36, and KvDSM 17-98 mutants (20<BSRad≤40). In addition, a middle tolerance with respect to a tolerant wild-type strain was observed in KvDSM 9-45, KvDSM 12-53, KvDSM 17-87, KvDSM 9-31, KvDSM 15, KvDSM 18 and KvDSM 21 because their BSR values ranged from 40 to 60 (Table 3); this implies a decrease in the BSR depending on the tested mutant strain.
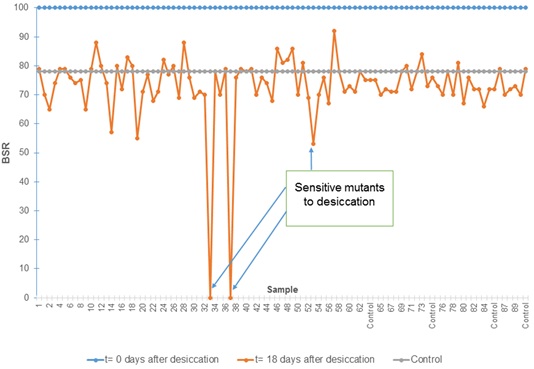
Strain | BSR 18 days After Desiccation | Related Protein |
---|---|---|
KvDSM 1-29 | 39.04 ± 8.2 | Flavin-containing monooxygenase FMO |
KvDSM 9-20 | 39.84 ± 3.1 | Nitrogenase molybdenum-iron protein beta chain (nifK) |
KvDSM 9-31 | 48.14 ± 2.2 | TraL conjugation Plasmid pSG1 |
KvDSM 9-45 | 53.24 ± 4.8 | Acetyltransferase gnat family |
KvDSM 12-43 | 21.86 ± 5.9 | Dihydroorotase |
KvDSM 12-53 | 42.82 ± 6.1 | Putative secretion ATPase |
KvDSM 12-93 | 6.62 ± 5.1 | Putative secretion ATPase |
KvDSM 17-36 | 37.69 ± 8.9 | Dihydroorotase |
KvDSM 17-87 | 45.93 ± 0.9 | Exported solute-binding protein |
KvDSM 17-98 | 37.23 ± 10.2 | Exported solute-binding protein |
KvDSM 6 | 0 ± 0.5 | BetR domain protein |
KvDSM 15 | 56.06 ± 15.2 | Conserved domain protein |
KvDSM 18 | 51.54 ± 8.3 | CadC operon activator |
KvDSM 21 | 44.39 ± 5.6 | Arabinose operon regulatory protein |
K. variicola T29A (wild type) | 68.48 ± 9.3 | – |
Each value represents the average of 5 repetitions. The values of the standard deviation are indicated.
3.3. Transposon Insertion Sites in Mutants of K. variicola T29A: Mini-Tn5Km
Genomic DNA of the 14 selected mutants and wild-type strain was used to amplify the kanamycin-resistance gene to corroborate the insertion of the mini-Tn5 transposon in the genome of the mutants sensitive to desiccation. As expected, the 1 kb amplification product was only observed in the mutants and not in the wild-type strain (data not shown).
To identify the genes disrupted by the transposon, an arbitrary PCR method was carried out in the desiccation-sensitive mutants. As described above, in this method, 2 rounds of PCR were carried out, allowing the region to be amplified from the terminal sequence of the kanamycin resistance gene near the “O” region that delimits the transposon and part of the interrupted gene. The amplification products were separated by agarose gel electrophoresis, where 200 to 600 bp sized bands were observed for each of the 14 mutants, however, in the wild-type strain used as a control, there was no amplification (Supplementary Fig. 3). The miniTn5-Km transposons of mutants with the lowest BSR values were inserted into genes related to BetR domain protein (KvDSM 6), putative secretion ATPase (KvDSM 12-93) and dihydroorotase (KvDSM 17-36) (Table 3).
3.4. Amplification of the betR Gene with Specific Oligonucleotides and Verification of its Mutagenesis in the Mutant KvDSM 6
PCR was performed to amplify the betR gene using oligonucleotides at the beginning and end as well as oligonucleotides specific to the mini-Tn5 transposon (Fig. 1). Products of 695 and 490 base pairs were obtained for the reactions carried out with the oligonucleotides Mut 6 stop-Fw/Tn5-Rv and Mut 6 start-Rv/Tn5-Fw, respectively. These amplification products corresponded to the fragments expected from both the beginning and the end of the betR gene, including fragments of the transposon that interrupted the gene. In the control reaction (with genomic DNA of the wild-type strain), no amplification product was obtained (Suppl. Fig. 4). Each amplification product was purified and sequenced. The obtained sequences were compared with the databases of the NCBI by BLAST, and it was verified that they corresponded to the gene that encodes the BetR protein. The partial sequences of the betR gene are shown in Fig. (1).
3.5. trans Complementation of Mutant KvDSM 6 with the betR Gene
The pJET1.2/blunt-BetR construct was incorporated by heat shock transformation into mutant KvDSM 6, which was interrupted in the betR gene. The incorporation of this construct was verified by comparing the plasmid profile of the mutant containing the miniTn5 inserted in the betR gene with respect to several transformants of the mutant complemented in trans with the pJET1.2-BetR construct. The presence of this construct was observed in these transformants, whereas in the KvDSM 6 mutant, it was not observed (Supplementary Fig. 5). The pJET1.2-BetR construct was purified from the complemented mutant, and double-stranded sequencing of the cloned fragment was performed using pJET1.2 forward and pJET1.2 reverse sequencing primers of the pJET1.2/blunt vector. From these sequences, the reconstruction of the cloned insert was performed and compared using BLAST in the NCBI database. Observations showed that it corresponded to the betR gene and did not undergo modifications in the genetic manipulation process. The complete sequence of the betR gene from the vector used for complementation was deposited into the GenBank database with the accession number MK085750.
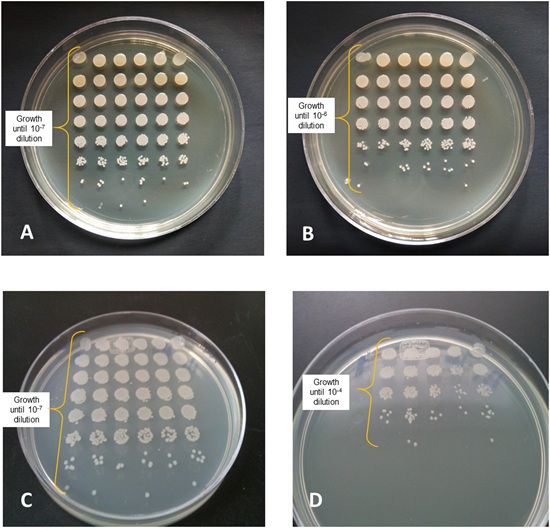
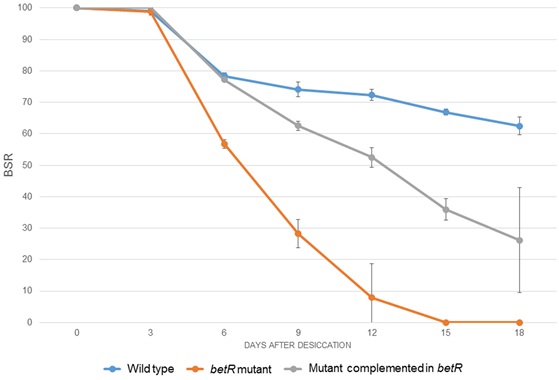
The complemented mutant was subjected to desiccation-rehydration tests together with the mutant and the wild-type strain. It was observed that at 18 DABD, the survival of the complemented mutant, (BSR value of 26.2), was greater than the mutant strain (BSR value of 0), but it does not reach the values observed for the wild-type strain, suggesting a partial complementation of the desiccation-resistance phenotype (Fig. 4).
4. DISCUSSION
Water is a determining factor for the life of living organisms since the vital processes of organisms depend mostly on the presence of water molecules to carry out the different chemical reactions in cells and maintain the cohesiveness of macromolecules and the interactions of cells with their surrounding environment [21, 31]. Throughout evolution, bacteria have faced changing environmental conditions, including the low availability of water in the environment, therefore those characteristics are selected that allow them to survive under stress conditions. Tolerance to desiccation has been evaluated for different bacterial strains, and findings show that some of them, such as K. variicola T29A, exhibit high levels of survival during these stress events [47]. Therefore, it was interesting to identify the genes involved in the tolerance to desiccation in this bacterial strain.
In this work, the generation of a mutant library and its subsequent evaluation of desiccation-tolerance allowed identification of 14 mutant strains that significantly decreased in their survival rates after desiccation. These mutants contained a mini-Tn5 transposon inserted into gene-encoding proteins related to different cell processes. Within the genes, mutant 1-29 was identified and inserted in a gene-encoding protein related to monooxygenase, an enzyme that has been linked to the biodegradation of toxic compounds and hydrocarbons in some bacteria [58, 59]. It is possible that the relationship of this enzyme with the resistance to desiccation could be associated with the degradation of secondary metabolites generated during stress. In Rhodococcus jostii RHA1, it has been observed that under dry conditions, there is an increase in the expression of genes related to monooxygenases [46]. Another mutant sensitive to desiccation-rehydration contained the mini-Tn5 inserted in the nifK gene, which codes for a subunit of the nitrogenase enzyme [60-62], which has the capacity to reduce molecules with triple bonds such as acetylene and cyanides [63]. It is possible that compounds with triple bonds are formed during desiccation, which can be toxic to the cell, as in the case of the Maillard reactions [21]; therefore, the role of the nitrogenase enzyme in the resistance to desiccation-rehydration could be related to the elimination of those compounds. Another mutant with decreased tolerance to this stress was inserted in the traL gene, which is part of an operon that is responsible for the transfer of genetic material through pili formation; traL gene has been linked to the pilus assembly (possibly a peripheral membrane protein) [64]. The tra genes also allow the adhesion of bacterial cells to guarantee the transfer of genetic material [64, 65]; traN has been suggested as a key factor in bacterial aggregation [66-69], an important step in the formation of biofilm, which is a bacterial mechanism to tolerate desiccation [70-72]. In the process of cell aggregation for the tolerance of different stress conditions; the production of exopolysaccharides plays an important role; in this study, a mutant was inserted in a gene that codes for a regulatory protein of the arabinose operon; this monosaccharide is present in the exopolysaccharides of some bacteria [73, 74], and it could be closely related to desiccation tolerance.
In the present work, the mutant containing the mini-Tn5 inserted in the betR gene was selected to perform complementation with the wild-type gene because its survival was null after 18 days of desiccation stress. After being complemented in trans with the betR gene, the desiccation tolerance of K. variicola mutant KvDSM 6 was significantly increased with respect to the mutant, suggesting that the protein encoded by this gene is important for the survival of K. variicola T29A under desiccation conditions. Complementation in trans was unable to restore tolerance to desiccation at the level of the wild type strain; the expression level of the betR gene could affect the modulation of genes involved in the synthesis of betaine. Because pJet 1.2/blunt cloning vector has a relatively high copy number (10-15 per cell), the expression levels of betR in the complemented strain could be increased compared to the wild-type strain, affecting fine modulation as observed for other complemented genes [75]. It has been determined that the betR gene encodes a transcriptional regulator of the LysR family, called BetR, that participates in the regulation of the transport and the metabolic process of choline-O-sulfate, which is used by the cell as a carbon or sulfur source or as a precursor for the synthesis of glycine betaine [76-78], a compound that has been linked to osmoprotection in different bacteria [79, 80]. Mechanism of betaine production has been studied only in some bacteria like Pseudomonas putida, Ensifer meliloti, Bacillus subtillis, Halomonas elongata DSM 3043 and Pseudomonas sp. ATCC19151 [76-78, 81, 82]; however, for Klebsiella variicola no study has been reported. Future work will be important to elucidate complete mechanisms related to betR gene. Desiccation is an event occurring frequently in the environment and bacterial strains must have several ways to confront this stress. The elucidation of mechanisms involved in desiccation-tolerance could allow us to formulate inoculants containing beneficial bacteria with these mechanisms or engineered beneficial bacteria where these characteristics were inserted. These kinds of bacteria will be more competitive and prominent to promote the growth of plants under water-stressed environments.
CONCLUSION
In the present work, some gene-encoding proteins involved in desiccation tolerance in K. variicola T29A were identified using a transposon mutant library, establishing a relationship between the insertion site of the transposon and the function of the interrupted gene. This knowledge is the basis for the understanding of the molecular mechanisms of bacteria to survive desiccation. BetR is involved in the tolerance of K. variicola T29A to desiccation, and its function could be analyzed in other beneficial bacteria with this protein. Alternatively, the betR gene could be introduced in other beneficial bacteria to explore the role of the encoded protein in these genetic backgrounds.
ETHICS APPROVAL AND CONSENT TO PARTICIPATE
Not applicable.
HUMAN AND ANIMAL RIGHTS
No animals/humans were used for studies that are the basis of this research
CONSENT FOR PUBLICATION
Not applicable.
AVAILABILITY OF DATA AND MATERIALS
Not applicable.
FUNDING
Apoyo Redes PRODEP 2015-2016 (CA-262 and CA-244), DITCo2016-3, DITCo2016-4 and VIEP-BUAP-2018 (100425 788-VIEP2018).
CONFLICT OF INTEREST
The authors declare no conflict of interest, financial or otherwise.
ACKNOWLEDGEMENTS
Osvaldo Rodríguez-Andrade received a CONACYT fellowship. We are also grateful to Ángeles Pérez Oseguera for the advice provided in the process of cloning the betR gene. We are grateful to Estrella Duque and Juan Luis Ramos for being the receptors of Andrés Corral-Lugo (Beca Mixta CONACYT) to perform massive desiccation experiments. Thanks to Lucía López-Reyes for providing the strain K. variicola T29A and for discussion about this work.
SUPPLEMENTARY MATERIAL
Supplementary material is available on the publishers Website along with the published article.