All published articles of this journal are available on ScienceDirect.
Estimation of Biofilm Components and Prevalence of SDR Genes among Staphylococcus Aureus Isolated from Anbar Hospitals
Abstract
Background
Nosocomial infections, a major health problem, are due at 80% to biofilm‐associated infection. Staphylococcus aureus, a prominent biofilm producer, has both MRSA and MSSA biofilm-forming capabilities that highlight its persistence in hospital environments. In previous studies, less focus was given to the components of the biofilms. Therefore, understanding biofilm composition has become crucial.
Objective
In this study, the components in the biofilm matrix in MRSA and MSSA isolates were estimated, and the prevalence of sdr genes (sdrC,sdrD,sdrE) among S. aureus strains was investigated.
Methods
Between September, 2023 and January, 2024, a total of 200 specimens were collected from patients with suspected Staphylococcus aureus infections. Eighty-two isolates were identified as S. aureus from various sources, including wounds, nasal swabs, ear swabs, and blood, based on macroscopic, microscopic, and molecular properties. Biofilms were subjected to DNase and proteinase K treatment in order to identify their biochemical composition. Multiplex PCR was used to determine the distribution of sdr genes among isolates.
Results
The average reduction after treatment with proteinase K in MSSA biofilms (29.67±16.62) was significantly lower than in MRSA biofilms (46.21±26.33, p=0.032). Also, when treated with DNase, MRSA biofilms showed a greater average reduction (49.65±25.30) compared to MSSA biofilms (28.71±20.98, p=0.006). MRSA and MSSA biofilms also had different polysaccharide concentrations, with MSSA biofilms having significantly higher levels (206.86±82.92 μM/OD595) than MRSA biofilms (85.80±32.52 μM/OD595, p=0.005). The sdrC gene was most common in the gene profiles of MRSA (100%) and MSSA (50%). There was a strong association between the presence of the sdrD gene and methicillin-resistant S. aureus isolates (p < 0.001).
Conclusion
These findings indicate that the biofilms of MRSA isolates are largely composed of proteins and eDNA, while MSSA isolates rely on polysaccharides in their biofilms. The results indicate the importance of proteins encoded by SDR genes in the development of biofilm structure.
1. INTRODUCTION
Staphylococcus aureus is an opportunistic pathogen that can lead to several self-limiting, even life-threatening dis- eases in humans [1]. S. aureus continues to be a significant contributor to hospital-acquired infections, even with the use of antibiotics and advancements in medical treatment [2].
One of the main reasons S. aureus infections are particularly problematic is the biofilm formation [3]. Biofilms are bacterial communities that produce a robust extracellular matrix of eDNA, protein, and poly- saccharides, serving as their first line of self-protection [4]. Currently, researchers generally believe that more than 80% of chronic infections are mediated by bacterial biofilms [5]. Attachment to human matrix proteins is the initial stage of biofilm formation [6]. S. aureus expresses numerous MSCRAMMs (microbial surface components recognizing adhesive matrix molecules) capable of binding to proteins like fibrinogen or fibronectin and often binding to multiple types of matrix proteins [6].
S. aureus carries genes called sdrC, sdrD, and sdrE. These genes are sited together in a specific region of its DNA known as the sdr locus. They code for proteins named serine-aspartate repeat proteins (sdr). The sdr proteins are members of the MSCRAMM family, of approximately 2.8, 3.9, and 3.5 kbp, respectively, located in the sdr locus. sdr proteins are not closely related, with only 20 to 30% identical amino acid residues, indicating that different sdr proteins have different roles in S. aureus pathogenicity [7]. The function of sdr proteins in S. aureus remains unknown. However, there have been a few studies that reported a strong correlation between sdr genes of S. aureus and certain human diseases according to the distributional assay of sdr genes [8]. These Sdr proteins are found on the surface of the bacteria and have a unique feature: a region rich in serine-aspartate pairs encoded by the variable number of repeats within the sdr genes [9]. This self-association of the serine-aspartate repeat protein sdr promotes both bacterial adherence to surfaces and biofilm formation [10]. This study aimed to understand whether sdr proteins play a role in biofilm formation by comparing their presence in strong, medium, and weak biofilms.
Understanding the composition of biofilms and the genes/proteins involved in their formation is crucial for breaking down existing biofilms or preventing their development [11].
Further research on the genes contributing to biofilm formation and the variations in biofilm composition among strains is crucial for advancing therapeutic developments, given the current limited understanding of Staphylococcus aureus biofilm structure and growth mechanisms [12]. Our study compared biofilm production by MRSA and MSSA strains isolated from patients and examined the prevalence of sdr genes among S. aureus strains.
2. METHODS AND MATERIALS
2.1. Sample Collection
This study was carried out in the College of Science, University of Anbar, Anbar, Iraq, in 2024. Over a period of approximately 4 months, the period from September, 2023 to January, 2024, 200 samples were collected from patients suffering from various infections suspected of being infected with S. aureus who visited “Fallujah Teaching Hospital, Women and Children's Hospital, Ramadi Teaching Hospital, and Ramadi Hospital for Women and Children”, as well as from patients who were admitted to different hospitals. The sources of these samples were different, including wounds, nasal swabs, blood, and ear swabs.
2.2. Identification of S. aureus Strains and Characterization
The samples were cultured immediately after collection for diagnosis, and S. aureus isolates were identified through cultural tests, microscopic diagnosis, biochemical tests, VITEK system diagnosis, and genetic diagnosis. A combination of tests was used to identify 82 isolates, such as S. aureus. These examinations included growth and fermentation on a selective medium (mannitol salt agar), cell type determination by gram staining, and catalase and coagulase tests for further confirmation. PCR amplification of the nuc gene also verified the isolates as S. aureus. Additionally, cefoxitin susceptibility testing and PCR amplification of the mecA gene were used to identify the isolates as either MRSA or MSSA [13, 14]. The work steps used in this study are shown in Fig. (1).
2.3. Antibiotics Susceptibility Test
The antibiotic susceptibility test was performed following CLSI guidelines 2023 [15]. Various classes of antibiotics were used in the antibiotic susceptibility test, which was carried out on 82 bacterial isolates by the disk diffusion method, as described previously [16]. Fifteen antibiotics (disks) tested against S. aureus were penicillin (10µg), ciprofloxacin (5µg), azethromycin (15µg), chloramphenicol (30µg), tetracycline (10µg), vancomycin (30µg), nitrofurantions (100µg), gentamycin (120µg), oxacillin (10µg), amoxicillin (10µg), doxycyillin (10µg), sulfamethoxazole (25µg), cefixime (5µg), amikacin (30µg), and cefoxitin (30µg) manufactured by Bioanalyse Company (Turkey origin).
2.4. Detection of Biofilm Formation
Biofilm formation was assessed semi-quantitatively using 96-well flat bottom plates, following established methods [17]. Bacterial inoculation was prepared by diluting bacteria in TSB with 1% glucose at a 1:100 ratio. Each well of a 96-well flat-bottomed polystyrene plate received 200 μL of bacterial suspension and then incubated overnight at 37°C. After incubation, plates were washed with PBS and stained with 0.1% crystal violet for 15 minutes at room temperature. Excess stain was removed by washing, and biofilm was quantified by measuring OD570 nm after solubilization in ethanol. Biofilm assays were performed in triplicate, and isolates with OD570 values ≥ the positive control were classified as biofilm-positive.
2.5. Effect of Protease K and DNase on Biofilm Structure
Biofilm composition was analyzed according to the previously described method [18]. After overnight growth in a 96-well plate, the remaining bacterial cells were removed, and wells were washed with PBS. Enzymatic treatments with proteinase K (100 μg/mL in 10Mm Tris-HCl buffer) and DNase (140 μg/mL in TSB) or a mock control were applied for 2 hours at 37°C. After washing, biofilms were fixed, stained, and eluted. OD595 nm was measured to calculate biomass formation inhibition percentage using the following formula:
(Percentage inhibition = 100 - ((OD595 nm experimental well with test material / OD595 nm control well without test material) x 100).
2.6. Extraction of “Extracellular Matrix (ECM)”
Isolates were cultured overnight in TSB at 37°C with shaking (200 rpm). After 16 hr. of incubation, cultures were diluted 1:200 in 50% TSB with 0.5% glucose and transferred 200 μL per well into 96-well plates (triplicate for each isolate). Biofilms formed overnight at 37°C without shaking were washed with distilled water, scraped, and suspended in 50μL of 1X PBS. After centrifuging at 8000 g for 10 minutes, pellets were resuspended in 1.5 M NaCl, centrifuged again at 5000g for 10 minutes, and the supernatant was collected for ECM analysis.
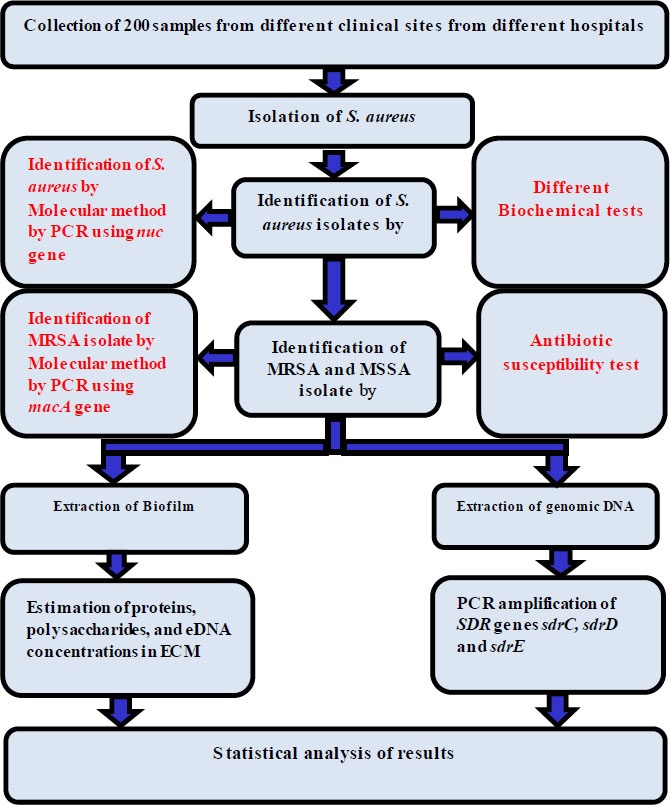
The work steps used in this study.
Primer Name | Primer Sequence 5′→3 | Estimated Product Size (bp) | Annealing Temperature (°C) | Source |
---|---|---|---|---|
nuc | F 5- GCGATTGATGGTGATACGGTT R 5-GCCAAGCCTTGACGAACTAAAGC | 270 bp | 61(C˚) | This study |
mecA | F 5-TCCAGATTACAACTTCACCAGG R 5- CCACTTCATATCTTGTAACG | 162 bp | 52(C˚) | This study |
sdrC | F 5- AAAAGGCATGATACCAAATCGA R 5- AATTCTCCATTCGTATGTTCTG | 144 bp | 55(C˚) | This study |
sdrD | F 5-AGTGGGAACAGCATCAATTTTA R 5-GTGGTAGATTGTACACTTTCTT | 272 bp | 55(C˚) | This study |
sdrE | F 5-AGAAAGTATACTGTAGGAACTG R 5-GATGGTTTTGTAGTTACATCGT | 433 bp | 53(C˚) | This study |
2.7. Estimation of “Proteins, Polysaccharides, and eDNA Concentrations in ECM”
The extracted ECMs were analyzed for biofilm protein concentration using the Bradford assay, following the method outlined previously [19]. The principle of this assay is that the binding of protein molecules to Coomassie dye under acidic conditions results in a color change from brown to blue. This method measures the presence of the basic amino acid residues, arginine, lysine, and histidine, which contributes to the formation of the protein-dye complex, allowing for relative concentrations of the purified protein to be determined. Polysaccharide concentration was assessed using the phenol-sulfuric acid assay following the method described previously [20]. The phenol-sulfuric acid method is a simple and rapid colorimetric method to determine the total carbohydrates in a sample. This method detected virtually all classes of carbohydrates (mono-, di-, oligo-, and polysaccharides). Results were analyzed by comparing the variation in OD595 units between untreated biofilms and DNase, proteinase K-treated biofilms, correlating with DNA or protein concentrations.
2.8. DNA Extraction
Isolates of S. aureus were grown on mannitol agar for a whole night. Three to four colonies were incubated at 37°C suspended in three milliliters of sterile distilled water. DNA was extracted using the Norgen-Canada Microbiome DNA isolation kit, and DNA was kept at -20°C.
2.9. Detection of sdr Genes
Multiplex PCR was performed to amplify the sdrC, sdrD, and sdrE genes, generating fragments of 144 bp, 272 bp, and 433 bp, respectively [21] (Table 1). PCR mixtures (50 μL) were prepared with 2 mM MgCl2 and 0.5μM each of forward and reverse primers. Moreover, 250μM each of dNTPs, 1.25 U of Taq DNA polymerase, 5 μL of 10X Taq buffer with KCl, and 50 ng of DNA template were also used. Amplification was conducted in a Thermocycler (T100; Biorad, Hercules, CA, USA) with 30 cycles of denaturation at 92°C for 45 seconds, annealing at 52°C for 30 seconds, and elongation at 72°C for 1 minute, followed by a final extension step at 72°C for 7 minutes [22]. The gene-specific PCR amplicons were visualized using Image Lab Software (Bio-Rad).
3. RESULTS AND DISCUSSIONS
3.1. Sample Collection and S. aureus Identification
Eighty-two isolates from 200 clinical specimens were diagnosed to be S. aureus based on biochemical testing, cultural and microscopical characteristics, automated Vitek2 system, and molecular identification by the nuc gene, which was collected from the hospitals mentioned in the methods. The main source of isolates was obtained from pus swabs obtained from patients admitted to the burn unit of the government hospital (wound 48 (58.53%), nasal swabs 18 (21.95%), blood 10 (12.19%), and ear swab 6 (7.3%)). All bacterial isolates (100%) with S. aureus were identified molecularly using the nuc gene (Fig. 2). The most frequent isolation from wounds was S. aureus, which was consistent with other earlier reports and other research, particularly those from wealthy countries, that identified S. aureus as the prevalent bacterium [23, 24]. In another study in Iran, S. aureus was found to be a common pathogen in wound infections at a rate of 20.2% [25]. Results of previous studies [26-28] have reported that both S. aureus and P. aeruginosa are the most frequently isolated bacteria in wound injuries. S. aureus is frequently found in wounds because it colonizes human skin and mucous membranes and can be transmitted from hands and nose to wounds. Burn wounds offer an ideal environment for bacterial growth and are more persistent sources of infection compared to surgical wounds, largely due to their larger size and extended hospital stays. Extensive wounds can also lead to immunosuppression [29].
3.2. Antibiotic Susceptibility Profiles
The highest resistance was reported to penicillin (100%), cefoxitin (83%), vancomycin (0%), oxacillin (83%), amoxicillin (76%), azithromycin (61%), tetracycline (26%), doxycycline (34%), sulfamethoxazole (16%), chlor- amphenicol (15%), ciprofloxacin (7%), nitrofurantoin (27%), gentamycin (0%), cefixime (100%), and amikacin (0%) (Fig. 3). Of the isolates, 14 (17%) sensitive isolates and 68 (83%) resistant isolates emerged. Such findings are in agreement with the previous study, which observed that about 86.04% of isolates were MRSA while 13.95% were MSSA [30]. This study found that 100% of the isolates were resistant to penicillin and 83% to oxacillin; both are β-lactam antibiotics that inhibit the production of cell walls. The overuse of these antibiotics may be the cause of this high resistance rate. These findings are consistent with those of a previous investigation [31], which found that S. aureus isolates from clinical hospitals in Cairo had 100% resistance to oxacillin and penicillin, which was also consistent with the results of other studies [32, 33]. For cefoxitin, this resistance is caused by two mechanisms: first, the expression of β-lactamase enzyme that hydrolyzes the β-lactam ring makes the antibiotic inactive, and second, the specific staphylococcal SCCmec (Cassette chromosome mec), which carries gene mecA that encodes for a protein called penicillin-binding protein (PBP2a) and has a poor tendency (affinity) for attaching β-lactams by substituting the endogenous PBP enzyme, which is a target of the antibiotic, makes the synthesis of the cell wall remains active. Moreover, the growth of S. aureus is also not affected due to its resistance to inhibition by β-lactams, thereby making the S. aureus resistant to a vast number of antibiotics belonging to β-lactams (such as cefoxitin) and also penicillin. This explains the cause of multi-antibiotic resistance in these strains. Resistance to these antibiotics occurs by different mechanisms, such as chemical modification, ribosomal protection, and efflux pump [34].
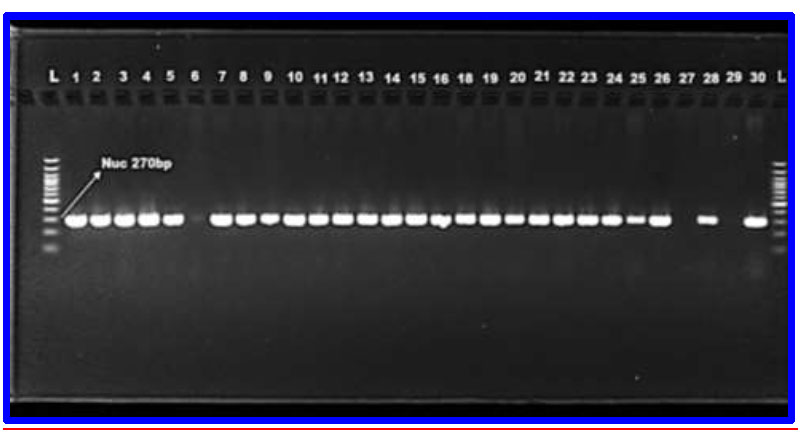
PCR identification of S. aureus isolates on the basis of the “nuc gene, which was amplified using the uni-plex PCR method. M(bp): 1.5% agarose, TAE buffer (1x), DNA ladder (100 bp), 70 volts for 1.5 hours”.
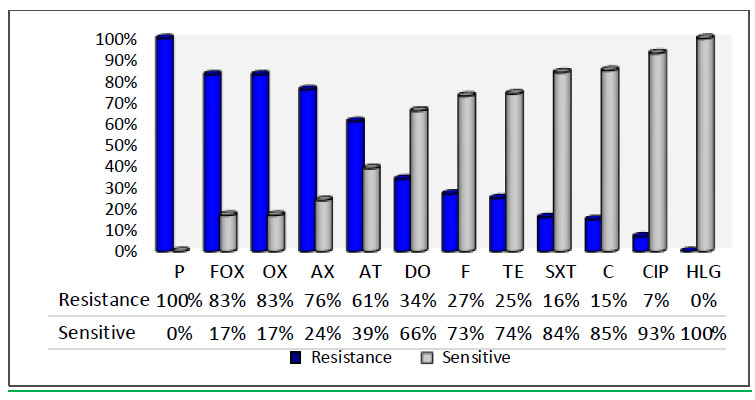
The percentage of antibiotic susceptibility test of S. aureus isolates. Penicillin (P), Cefoxitin (FOX), Oxacillin (OX), Amoxicillin (AX), Azithromycin (AT), Doxycyillin (DO), Nitrofurantoin (F), Tetracycline (TE), Sulfamethoxazole (SXT), Chloramphenicol (C), Ciprofloxacin (CIP), Gentamycin (HLG), Vancomycin(V), Amikacin (AK).
3.3. Biofilm Formation
Biofilm form of MRSA and MSSA isolates was measured by CV staining after overnight growth. MRSA biofilms are known to be more robust than MSSA biofilms [35, 36]. Biofilm mass showed significant variation in both MRSA and MSSA isolates. The mean OD595 for MSSA isolates was 0.37±0.22. However, the mean OD595 for MRSA isolates (0.78±0.46) was significantly higher, indicating more robust biofilm formation (p = 0.005). Understanding the matrix's organization and development is crucial for effective control strategies. Biofilms from each isolate were tested for exposure to degradation by proteinase K and DNase to determine their protein and eDNA content, respectively. Polysaccharide concen- trations were measured using the phenol sulfuric-acid assay on extracted ECMs. Biofilms of sensitive isolates were not significantly affected by proteinase K treatment, while biofilms of resistant isolates showed a significant reduction. The average reduction in MSSA biofilms (29.67±16.62) was significantly lower than in MRSA biofilms (46.21±26.33) (p=0.032), indicating higher protein content in MRSA biofilms. Protein concentrations from ECMs, estimated using the Bradford assay, showed a significant positive correlation with decreased crystal violet binding after proteinase K dealing (R2=0.0924, significance (p=0.040) (Fig. 4). Most MRSA and MSSA isolates were moderate biofilm producers, which was consistent with the findings of Smith et al. (2008) [37]. Controlling biofilms may focus on dispersing or degrading the extracellular polymeric matrix rather than directly killing the bacteria. MRSA isolates formed more robust biofilms with higher protein content compared to MSSA isolates. These findings are consistent with the results obtained from previous studies [38]. Dakheel et al. (2016) also identified proteinaceous components as key elements of the MRSA biofilm matrix [39]. It has been suggested that MSSA biofilms primarily consist of polysaccharides, whereas MRSA biofilms are composed mainly of DNA and proteins. The results indicated that S. aureus (MRSA) isolates have more protein-based biofilms. When the MRSA isolates were treated with proteinase, there was a high rate of biofilm reduction. This increase in the rate of biofilm reduction indicates a high concentration of proteins in MRSA isolates.
Similar to proteinase K, DNase treatment resulted in varying degrees of biofilm reduction for both MSSA and MRSA isolates (Fig. 5A-B). The average biofilm reduction for MRSA isolates (49.65±25.30) was significantly higher than for MSSA isolates (28.71±20.98) (p = 0.006), indicating that MRSA biofilms generally contain more eDNA. Previous studies have reported that strong biofilm producers of MRSA have higher eDNA levels compared to weak biofilm producers [40]. This indicates that eDNA plays a greater role in MRSA, with strong biofilm producers generally having more eDNA compared to MSSA isolates, which produce weak biofilms. eDNA is a crucial component of biofilm building [41]. DNase I treatment has been reported to block or alter biofilm formation in Gram-positive cells, such as S. aureus, S. pneumonia, and L. monocytogenes [41-43]. According to our data, DNase I significantly affected the dissemination of biofilms in the majority of the tested isolates. This result is in line with the findings reported by Rice et al. [44], who found that the structural stability of S. aureus biofilms relies on eDNA. DNase I-induced eDNA degradation reduces the biofilm [45]. Moreover, it has been found that eDNA not only increased biofilm stability but also its resistance to antibiotics. DNase I can prevent biofilm formation in both PIA-dependent MSSA and PIA-independent MRSA, as well as disperse preformed biofilms [46]. Other studies have focused primarily on protein and polysaccharide composition rather than the eDNA component of biofilms [47]. These results suggest that eDNA is generally necessary for the formation of large biofilms in MRSA. Previous studies reported that some low biofilm producers can have high levels of eDNA, indicating that eDNA quantity does not always correlate with biofilm-forming ability [48]. In contrast, this study reported that isolates MS1, MS3, MS9, MS10, MS11, MS12, MS13 (MSSA), and MR8 (MRSA), which produced weak biofilms, contained small amounts of eDNA. This suggests that eDNA levels correlate with biofilm-forming capacity. Targeting eDNA could be a key strategy for developing eradication methods against S. aureus biofilms.
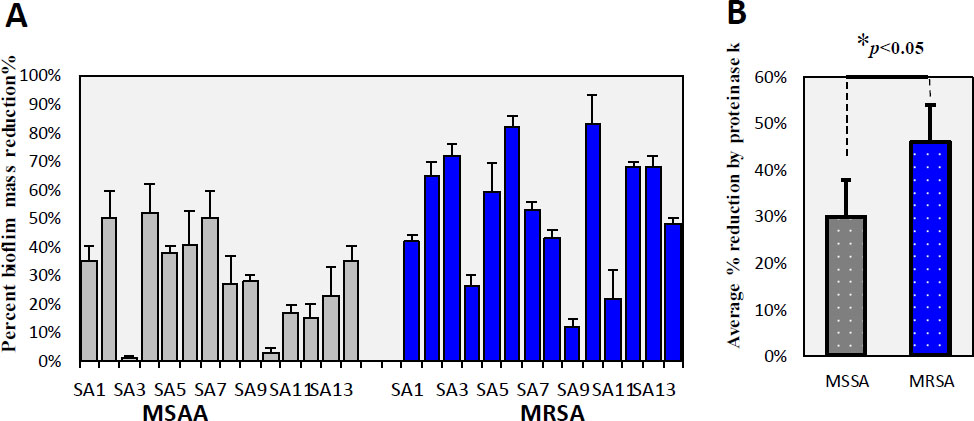
Effect of Proteinase K on biofilm structure in MSSA and MRSA Isolates: (A) Percent reduction in biofilm for MSSA (light color) and MRSA (dark blue) isolates. (B) Average percent reduction in biofilm for MSSA and MRSA isolates (p < 0.05).
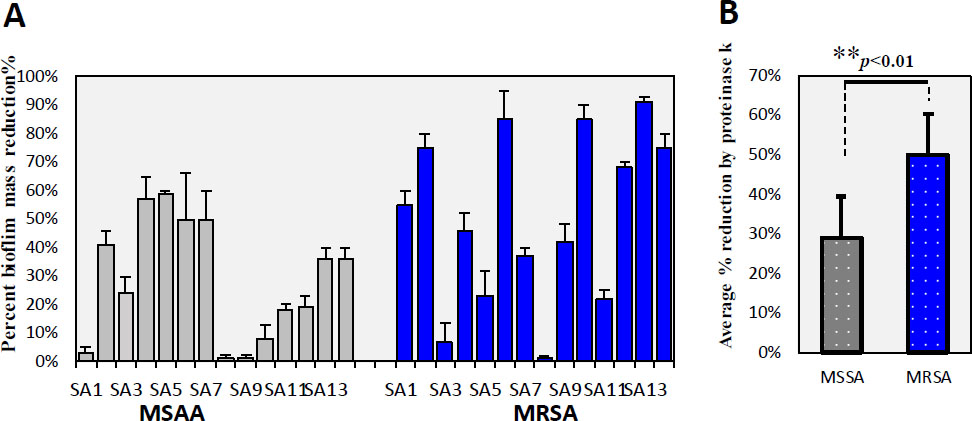
Effect of DNase on biofilm structure in MSSA and MRSA Isolates: (A) in biofilm for MSSA (light color) and MRSA (dark blue) isolates. (B) Average percent reduction in biofilm for MSSA and MRSA isolates (p < 0.01).
MRSA and MSSA exhibited differing polysaccharide content in their biofilms. MSSA biofilms had a significantly higher average polysaccharide concentration (206.86± 82.92 μM/OD595) compared to MRSA biofilms (85.80±32.52μM/OD595, p=0.005), indicating that MSSA biofilms contain more polysaccharides. O’Gara's group noted that methicillin-sensitive S. aureus (MSSA) typically forms biofilms dependent on PIA, whereas methicillin-resistant S. aureus (MRSA) tends to produce PIA-independent biofilms to a greater extent than MSSA [49].
3.4. Molecular Detection of sdr Genes among S. aureus Clinical Isolates
The serine-aspartate repeat proteins (sdr) are crucial surface proteins in S. aureus, contributing to its pathogenicity. Among 82 S. aureus isolates, 77 (93.90%) tested positive for at least one of the three sdr genes: sdrC (93.90%), sdrD (60.97%), and sdrE (79.26%). Specifically, 69 isolates (84.14%) were positive for two or three sdr genes. Notably, the prevalence of sdrC (100%), sdrD (80%), and sdrE (90%) was highest among blood isolates, followed by wound isolates with sdrC (100%), sdrD (71%), and sdrE (82%) (Table 2, Fig. 6).
- | - | - | Source | - | - |
---|---|---|---|---|---|
Bio marker | Total positive |
Wound (n= 48) |
Nasal swab (n= 18) |
Blood (n= 10) |
Ear swab (n=6) |
nuc | 82/82(100%) | 48/48(100%) | 18/18(100%) | 10/10(100%) | 6/6(100%) |
mecA | 68/82(83%) | 47/48(98%) | 7/18(39%) | 10/10(100%) | 4/6(67%) |
sdrC | 77/82(93.90%) | 48/48(100%) | 14/18(78%) | 10/10(100%) | 5/6(83%) |
sdrD | 50/82(60.97%) | 34/48(71%) | 6/18(33%) | 8/10(80%) | 2/6(33%) |
sdrE | 65/82(79.26%) | 42/48(88%) | 11/18(61%) | 9/10(90%) | 3/6(50%) |
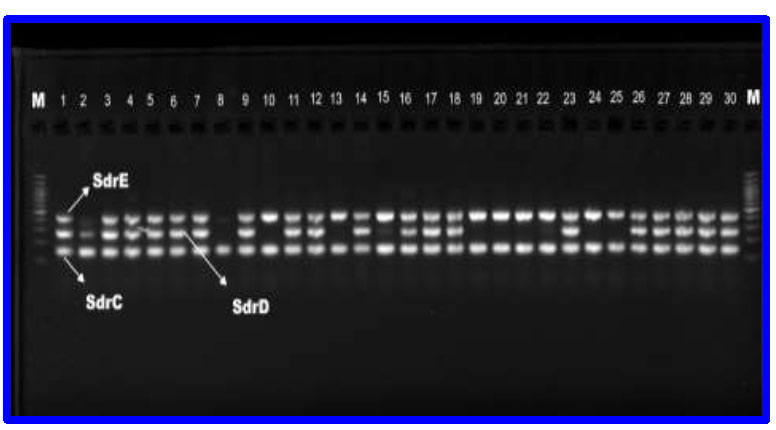
PCR products of Sdr genes. L ( Ladder 100-1200 bp), sdrC bands with 144bp, sdrD bands with 272pb, sdrE bands with 433bp.
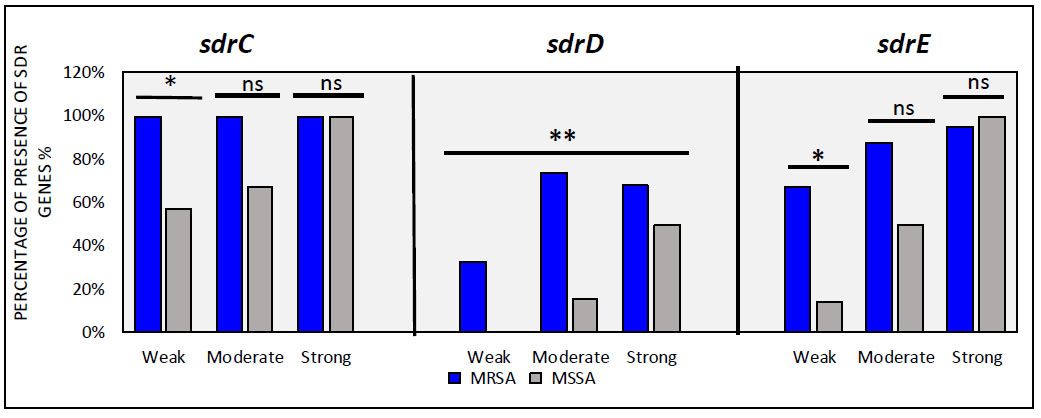
Prevalence of sdr genes among strong, medium, and weak biofilms in MRSA and MSSA of S. aureus isolates. asterisk (*) indicates significance at p < 0.05, (**) indicates significance at p < 0.01 and (ns) not significant.
3.5. Prevalence sdr Genes among S. aureus
Results of the prevalence of sdr genes among MRSA and MSSA isolates are presented in Table 3 and Fig. (7). Molecular detection revealed sdrC as the most prevalent gene (93.90%), followed by sdrE (79.26%) and sdrD (60.97%). Overall, sdr genes were more common in methicillin-resistant than methicillin-sensitive isolates. Notably, sdrC showed a significantly higher presence compared to sdrD and sdrE in both MRSA and MSSA isolates, which was consistent with previous findings [8]. In a previous study, all MRSA isolates were found to be positive for two or three (sdr) genes. Our findings indicate that the simultaneous presence of sdrC, sdrD, and sdrE genes together was higher in MRSA isolates (63.23%) compared to MSSA isolates (7%). Earlier research highlighted a significant association between the presence of the sdrD gene and MRSA isolates [7]. It was observed that the distribution of the SdrD gene was higher in MRSA isolates compared to MSSA isolates, often coexisting with both sdrC and sdrE genes rather than with just one of them. In contrast, the sdrC gene tended to coexist more frequently with sdrE (25%) than with sdrD (7.35%) in MRSA isolates. These findings highlight the prevalence of sdrD and sdrE genes in MRSA, suggesting their involvement in bacterial resistance and as significant virulence factors in resistant strains. A significant difference was observed in isolates between biofilm-strong producing and biofilm-weak producing with respect to the sdrC, sdrD, and sdrE genes. This result is consistent with the findings of several studies on sdrC in Staphylococcus aureus isolates from patients [7, 50, 51], where they observed that SDR genes are highly prevalent in invasive Staphylococcus aureus isolates compared to other types of SDR genes. This may indicate the importance of the protein in bacterial colonization and invasiveness of host tissues. In addition, considering the isolates as a whole, the presence of sdrD and sdrE significantly improved biofilm formation. Our study underscores the roles of sdrC, sdrD, and sdrE proteins in biofilm formation, particularly emphasizing the importance of sdrD in forming robust biofilms.
- |
S. aureus Isolates (n=82) (%) |
MRSA Isolates (n=68) (%) |
MSSA Isolates(n=14) (%) |
---|---|---|---|
sdrC | 77/82 (93.90%) | 68/68 (100%) | 9/14 (64%) |
sdrD | 50/82 (60.97%) | 48/68 (71%) | 2/14 (14%) |
sdrE | 65/82 (79.26%) | 61/68 (90%) | 4/14 (36%) |
sdrC+, sdrD+ and sdrE+ | 44/82 (53.65%) | 43/68 (63.23%) | 1/14 (7%) |
sdrC+, sdrD+ and sdrE− | 6/82 (7.31%) | 5/68 (7.35%) | 1/14 (7%) |
sdrC+, sdrD− and sdrE+ | 19/82 (23.17%) | 18/68 (25%) | 1/14 (7%) |
sdrC−, sdrD+ and sdrE+ | 0 | 0 | 0 |
sdrC+, sdrD− and sdrE− | 8/82 (9.75%) | 2/68 (3%) | 6/14 (42.85%) |
sdrC−, sdrD+ and sdrE− | 0 | 0 | 0 |
sdrC−, sdrD− and sdrE+ | 2/82 (2.43%) | 0 | 2/14 (14%) |
sdrC−, sdrD− and sdrE− | 5/82 (6%) | 0 | 5/14 (35.71%) |
+, positive; -, negative | - | - | - |
CONCLUSION
In conclusion, the virulence of S. aureus is largely attributed to its capability to form biofilms, which depends significantly on various proteins that facilitate this process. The estimation of biofilm components was studied, and the focus was on estimating proteins. The prevalence and distribution of the three sdrc genes (sdrC, sdrD, and sdrE) in MSSA and MRSA isolates were studied, and the prevalence in different biofilms (weak, moderate, and strong) was determined. This study reported the highest distribution of sdrC and sdrE genes in different biofilm formations. However, the sdrD gene showed the highest distribution level only in biofilms with high protein concentrations, highlighting the importance of encoded proteins in biofilm structure development and suggesting the role of sdrD in biofilm accumulation. However, further studies are needed to understand the exact role of sdrD in biofilm formation.
AUTHORS’ CONTRIBUTION
R. A. J.: Contributed to the data collection and analysis, writing the theoretical part and participating in the practical part under the full supervision of Professor S. A. L. for all work steps.
LIST OF ABBREVIATIONS
MSCRAMMs | = microbial surface components recognizing adhesive matrix molecules |
PBP2a | = penicillin-binding protein |
ETHICS APPROVAL CONSENT TO PARTICIPATE
The study was approved by the Al-Anbar Directorate of Health Ethics and Research Committee, Iraq (approval number 34, decision No. 2022054, dated October 25th, 2023).
HUMAN AND ANIMAL RIGHTS
All human research procedures followed were in accordance with the ethical standards of the committee responsible for human experimentation (institutional and national), and with the Helsinki Declaration of 1975, as revised in 2013.
AVAILABILITY OF DATA AND MATERIALS
The data supporting the findings of the article is available within the article itself.
FUNDING
Our research is self-funded, with the researchers bearing all costs. We do not receive financial support from our university, city, country, or any external sources.